Effects of propyl gallate on interaction between TNF-α and sTNFR-I using an affinity biosensor1
Introduction
Propyl gallate (PG) is a well-known synthetic antioxidant on the US Food and Drug Administration list (Figure 1). It is a derivative of gallic acid, which is one of the active ingredients of Paeoniae Radix, a commonly used traditional Chinese medicine (TCM). Besides its antioxidant activity, PG has many pharmacological effects such as free radical elimination[1,2], inhibiting platelet aggregation[3,4], anti-inflammatory effects[5] and anti-tumor activities[6]. It is used widely to nourish blood, activate circulation, alleviate pain, regulate menstruation and treat liver disease and cancer. However, until now the research on PG has been focused on its curative effects at the holistic level. There are no detailed studies on the mechanism and dynamics of action at the molecular level, which limits the further clinical use of PG.
Tumor necrosis factor-α (TNF-α) is produced mainly by activated macrophages and monocytes. Mature TNF-α molecules are released as 17 kDa monomers, under physiological conditions, and the monomers associate non-covalently to form a homotrimer that is biologically active[7,8]. TNF-α is one of the most pleiotropic cytokines; besides its antitumor activity in vitro and in vivo, it plays an important role in many physiological and pathophysiological responses, such as inflammatory reactions, thrombosis, cardiac failure, infection, immune regulation, antiviral responses and endo-toxic shock, as well as in the pathogenesis of certain autoimmune diseases[9–13].
The biological activities of TNF-α are mediated by binding to 2 distinct membrane TNF receptors (TNFR), TNFR-I (p55, ~55 kDa) and TNFR-II (p75, ~75 kDa). Although both TNFR-I and TNFR-II bind TNF-α with high affinity, it has been generally believed that most of the cellular TNF responses are dominated by TNFR-I. Membrane TNFR (mTNFR) can be cleaved proteolytically to release soluble forms of the receptors (sTNFR). Though sTNFR lacks an intracellular domain, it binds free TNF-α with high affinity, competing with mTNFR[14,15].
Though their physiological roles are not fully understood, sTNFR might regulate the function of TNF in vivo through 2 different mechanisms: (i) by eliminating mTNFR and thereby reducing cellular reactivity to TNF, sTNFR may protect the cell from TNF pathological injury; and (ii) whereas sTNFR inhibits the activity of TNF by binding and neutralizing the cytokines at high concentrations, it may also enhance TNF activity at low concentrations by stabilizing TNF trimer molecules and prolonging their availability for binding mTNFR, thereby acting as an immunoregulator[16,17].
Because sTNFR is present constitutively in serum derived from receptor shedding after cellular activation by stimuli such as TNF-α production[18], sTNFR serum level is considered a reliable indicator of TNF-α system activation[19]. Moreover, TNF-α and sTNFR are considered to be key disease molecules and therapeutic targets[9,20]. Because the pharmacological functions of PG and the biological activity of TNF-α are probably correlative, the TNF-α–mTNFR–sTNFR system may be a potential target of PG in vivo. In order to find out if this is the case, in the present paper, an affinity biosensor, the cuvette-based IAsys biosensor, was used to study the effects of PG on the interaction of TNF-α with sTNFR-I.
Materials and methods
Chemicals N-hydroxysuccinimide (NHS), 1-ethyl-3-(3-dimethylaminopropyl) carbodiimide (EDC), ethanolamine and PG were purchased from Sigma-Aldrich (St Louis, MO, USA). TNF-α and sTNFR-I were purchased from PeproTech EC (London, UK). Carboxymethyl dextran (CMD) dual-well cuvettes were purchased from Labsystems Affinity Sensors (Cambridge, UK). Phosphate-buffered saline Tween-20 (PBST, pH 7.4) was composed of 10 mmol/L Na2HPO4/NaH2PO4, 138 mmol/L NaCl, 2.7 mmol/L KCl and 0.05% Tween-20. All solutions were made by using deionized water. All reagents were of analytical grade and were used without further purification.
Apparatus All analyses were carried out using an IAsys Plus optical biosensor (Labsystems Affinity Sensors, Cambridge, UK), which is based on resonant mirror technology. This instrument employs a dual-well stirred cuvette (pre-derivatized with CMD) and monitors the interaction between a pair of biomolecules inside the reaction cuvette[21–23]. One of the biomolecules, the ligand, is immobilized to the sensing surface of the cuvette. Its binding partner, the ligate, is then injected into the cuvette. Changes in refractive index and thickness on the sensing surface occur upon binding (association) of ligate to the immobilized ligand. The IAsys biosensor detects these changes continuously, producing a plot of responses that are measured in arc seconds[24–27]. Following each interaction, the surface of the cuvette is regenerated to remove bound ligate, and another ligate can then be introduced to the sensing surface. The IAsys biosensor has been applied to many kinds of studies on molecular recognition, affinity, kinetics, solute concentrations and multi-molecular interactions. It is especially useful for capturing measurements quickly and in real time, and can eliminate the need for radiolabels or other chemical tags. Compared to traditional methods, numerous additional steps are saved.
sTNFR-I immobilization To examine the binding of TNF-α to sTNFR-I, the receptor protein was immobilized onto the cuvette surface through its residual amine groups via amide with CMD. The IAsys instrument parameters were set at 100% for stirring, and 0.3 s for the sampling interval. The running buffer was PBST. Data acquisition then began and baseline data was gathered for several minutes. The surface of the cuvette was activated with 40 µL of a 1:1 (v/v) EDC/NHS mixture (0.4 mol/L EDC and 0.1 mol/L NHS) for 7 min. The function of EDC/NHS is to activate and promote the formation of covalent linkages by forming the N-hydroxysuccinimide ester. The carboxyl on the CMD bound NHS under the action of EDC and created active ester intermediates that bound the primary amines of sTNFR-I to make the amide bonds. Thus, sTNFR-I can be covalently immobilized on the CMD. The cuvette was subsequently washed with PBST and 10 mmol/L acetate (pH 5.5). After gathering baseline data in the acetate buffer, 10 µL diluted sTNFR-I was added to the cuvette to begin the electrostatic uptake of protein and covalent coupling. After a sufficient reaction time, 15 min or so, unreacted NHS esters were blocked by 40 µL 1 mol/L ethanolamine (pH 8.5) and the baseline was stabilized by washing with PBST.
At the same time, another cuvette channel was used as the blank control, without anchoring of sTNFR-I. The acetate buffer was injected instead of the sTNFR-I solution.
Binding detection The sTNFR-I modified and unmodified cuvettes were both rinsed 3 times with 40 µL PBST, and 10 µL TNF-α (0.01 mg/mL) was then added into each of the cuvettes to equilibrate for 15 min, before they were washed with PBST. The resonant angle response was recorded. The chip surface was then regenerated by adding 10 mmol/L HCl, until the response signal returned to baseline to proceed with another binding cycle. To evaluate the affinity binding of TNF-α and sTNFR-I, a wide range of TNF-α concentrations (from 0.625 µg/mL to 20 µg/mL) were used.
Effects of PG on binding of TNF-α and sTNFR-I After 3 times of washes with 40 µL PBST, 10 µL TNF-α (0.01 mg/mL), preincubated with different concentrations of PG, was added into the sTNFR-I-modified cuvette. Binding between TNF-α and sTNFR-I was detected as described above.
Results
sTNFR-I immobilization on the surface of cuvettes As described in the “sTNFR-I immobilization” section, the sTNFR-I molecules were immobilized onto the surface of the IAsys cuvette by the ester exchange reaction of NHS to COOH groups on CMD. Figure 2 shows the response sensorgram of the IAsys biosensor to EDC/NHS activation as well as to the consequent sTNFR-I immobilization (solid line). Phase (1) is the initial baseline obtained when the surface of the cuvette was exposed to the PBST; phase (2) is the addition of EDC/NHS mixture to the sensor chip; phase (3) is the buffer washing to remove unreacted EDC/NHS mixture; phase (4) is the washing with acetate buffer; phase (5) is the addition of sTNFR-I; phase (6) is the dissociation course by washing with PBST; phase (7) is the blocking of non-coupled activated CMD sites with ethanolamine; phase (8) is the baseline stabilization. Arrow (9) is the resonant angle shift caused by the immobilization of sTNFR-I onto the cuvette surface. The broken line indicates the reference channel signal in order to follow non-specific binding on the biosensor surface.
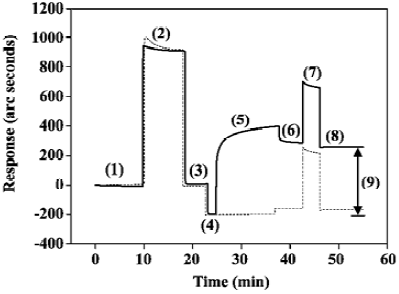
The amount of immobilized sTNFR-I was calculated by subtracting the baseline level after phase (4) from that after phase (8), described in Figure 2 as arrow (9), which is approximately 450.61 arc seconds. The sensitivity of the CMD cuvette is 163 arc seconds/ng per mm2. Thus the density of immobilized sTNFR-I is 2.76 ng/mm2. Dual-well IAsys cuvettes have a sensor area of 4 mm2, so the total amount of sTNFR-I immobilized was 11.04 ng.
Detection of interaction between sTNFR-I and TNF-α Figure 3 shows the comparison of TNF-α binding to the sTNFR-I modified cuvette surface (solid line) and the unmodified surface (broken line). The results showed that there was no specific binding on the unmodified cuvette surface except for non-specific absorption, which was easily washed away. This indicates that TNF-α bound sTNFR-I on the cuvette surface specifically.
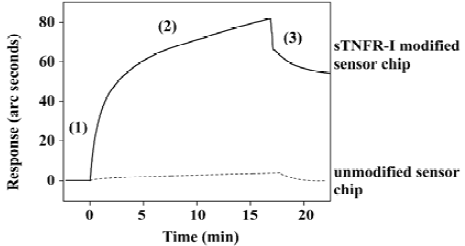
The dissociation equilibrium constant (KD) of binding of TNF-α and sTNFR-I were determined by using the FASTfit program, which is specifically designed for biomolecular interaction analysis using the IAsys biosensor. The KD value was 5.05×10-7 mol/L, while the KD of TNF-α/mTNFR-I binding has been reported as about 1×10-9 mol/L[28]. It can be inferred that though sTNFR-I can bind TNF-α with high affinity, its binding ability is lower than mTNFR-I. The results indicate that sTNFR-I binding affinity for TNF-α is decreased, which is probably attributable to the denaturation that occurred when sTNFR-I was produced.
Effects of PG on the interaction between TNF-α with sTNFR-I Figure 4 shows the experimental results obtained at a PG concentration of 500 nmol/L. With the presence of PG, the initial velocity of TNF-α/sTNFR-I binding increased and the time taken to approach equilibrium decreased, which indicated that PG was able to enhance the binding of TNF-α to sTNFR-I.
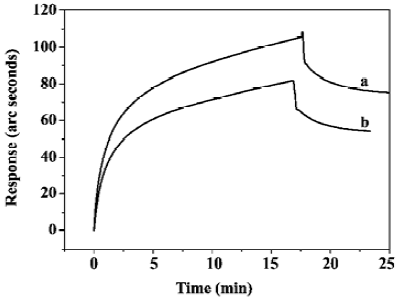
The concentration-dependent effects of PG on the binding of sTNFR-I and TNF-α were studied. The PG concentrations varied from 5 nmol/L to 500 nmol/L, and the results are summarized in Figure 5. The increase in amplitude of the initial velocity of TNF-α/sTNFR-I binding, as well as the decrease in amplitude of the time taken to approach equilibrium, both increased as PG concentration increased, which indicated that PG enhanced TNF-α/sTNFR-I binding in a dose-dependent manner.
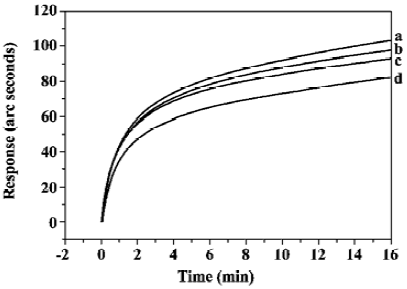
Discussion
Recent researches showed that the pathogenesis of the traditional Chinese medicine syndromes is the result of perturbation of cytokine networks, and the mechanism of TCM is to regulate and correct the perturbation of cytokine networks. It has been observed for many years that Chinese prescriptions can regulate the gene expression level of cytokines or their receptors. But for the initial step of the cytokine action, the binding to the corresponding receptor, there is few reports mainly due to the lack of appropriate methods. In the present study, an affinity biosensor, the IAsys biosensor, was used to explore the interaction of the cytokine and its receptor. The involvement of TNF-α in the pathogenesis of various diseases makes it an obvious and attractive therapeutical target. The effects of PG on the interaction between TNF-α and sTNFR-I were examined by using the IAsys biosensor in an in vitro assay. The experimental results showed that PG enhanced TNF-α/sTNFR-I binding in a dose-dependent manner. Though the mechanisms of PG are not clear and the roles of TNF-α and sTNFR-I are debated, it can be concluded that the binding between TNF-α and sTNFR-I is another target that PG can act on in vivo. Researches showed that serum level of sTNFR-I are often increased in a variety of conditions which are characterized by an antecedent increase in TNF-α, such as sepsis, inflammatory responses, autoimmune diseases, human immuno-deficiency virus infection, transplant rejection and malignant tumor[29–32], which indicated that sTNFR-I production seems to neutralize and reduce the toxicity associated with the elevated serum TNF-α level. PG can enhance the cellular protection of sTNFR-I through increasing neutralization to TNF-α, which is in agreement with the experimental and clinical results reported[33]. Thus it may sound theoretically that PG is helpful for therapy or assistant therapy in TNF-α corresponding diseases, which may leads to the new therapeutic application of PG. These are the first results obtained using affinity biosensors for studying the interactions of these molecules. This finding offers a new clue to the study of the mechanism of action of PG. The IAsys biosensor could be used widely as a reliable tool for similar studies. With respect to most of the other available methodologies to study biomolecular interactions, there are many advantages of this new method, such as real time measurement, high sensitivity, high selectivity and high veracity, as well as being easy to handle and eliminating the need for labeled compounds. It has tremendous application prospects in studies on the mechanisms of TCM in vitro. It will be more compellent when data on bioactivity, such as from cell experiments, were acquired to support the results from those from biosensor technology. Further studies in this area are in progress.
References
- Reddan JR, Giblin FJ, Sevilla M, Vanita P, Dorothy CD, Victor RL, et al. Propyl gallate is a superoxide dismutase mimic and protects cultured lens epithelial cells from H2O2 insult. Exp Eye Res 2003;76:49-59.
- Shanker G, Aschner M. Methylmercury-induced reactive oxygen species formation in neonatal cerebral astrocytic cultures is attenuated by antioxidants. Brain Res Mol Brain Res 2003;110:85-91.
- Panganamala RV, Miller JS, Gwebu ET, Sharma HM, Cornwell DG. Differential inhibitory effects of vitamin E and other antioxidants on prostaglandin synthetase, platelet aggregation and lipoxidase. Prostaglandins 1977;14:261-71.
- Chen KJ. Antithrombosis of propyl gallate. Chin Prescrip Drug 2003;9:38-9.
- Franzone JS, Natale T, Cirillo R. Influence of propyl gallate and 2-mercapto-propionyl glycine on the development of acute inflammatory reactions and on biosynthesis of PGE2. Bull Soc Ital Biol Sper 1980;56:2539-45.
- Lee SMY, Li MLY, Tse YC, Leung SCL, Lee MMS, Tsui SKW, et al. Paeoniae Radix, a Chinese herbal extract, inhibits hepatoma cells growth by inducing apoptosis in a p53 independent pathway. Life Sci 2002;71:2267-77.
- Carswell EA, Old LJ, Kassel RL, Green S, Fiore N, Williamson B. An endotoxin-induced serum factor that causes necrosis of tumors. Proc Natl Acad Sci USA 1975;72:3666-70.
- Smith RA, Baglioni C. The active form of tumor necrosis factor is a trimer. J Biol Chem 1987;262:6951-4.
- Taylor PC, Williams RO, Feldmann M. Tumour necrosis factor α as a therapeutic target for immune-mediated inflammatory diseases. Curr Opin Biotechnol 2004;15:557-63.
- Piguet PF, Vesin C, Chen DK. Activation of platelet caspases by TNF and its consequences for kinetics. Cytokine 2002;18:222-30.
- Guarntz D, Cowling RT, Vaiki N, Frikovsky E, Moore CD, Greenberg BH. IL-1β and TNF-α upregulate angiotensin II type 1 (AT1) receptors on cardiac fibroblasts and are associated with increased AT1 density in the post-MI heart. J Mol Cell Cardiol 2005;38:505-15.
- Zimmermann VS, Bondanza A, Rovere-Querini P, Colombo B, Sacchi A, Fascio U, et al. Characterisation of functional biotinylated TNF-á targeted to the membrane of apoptotic melanoma cells. J Immunol Methods 2003;276:79-87.
- Mocellin S, Rossi CR, Pilati P, Nitti D. Tumor necrosis factor, cancer and anticancer therapy. Cytokine Growth Factor Rev 2005;16:35-53.
- Mwangi SM, Stabel J, Lee EK, Kehrli ME, Taylor MJ. Expression and characterization of a recombinant soluble form of bovine tumor necrosis factor receptor type I. Vet Immunol Immunopathol 2000;77:233-41.
- Bao L, Lindgren JU, Zhu Y, Ljunggren HG, Zhu J. Exogenous soluble tumor necrosis factor receptor type I ameliorates murine experimental autoimmune neuritis. Neurobiol Dis 2003;12:73-81.
- Pennica D, Kohr WJ, Fendly BM, Shire SJ, Raab HE, Borchardt PE, et al. Characterization of a recombinant extracellular domain of the type 1 tumor necrosis factor receptor: evidence for tumor necrosis factor a induced receptor aggregation. Biochemistry 1992;31:1134-41.
- Aderka D, Engelmann H, Maor Y, Brakebusch C, Wallach D. Stabilization of the bioactivity of tumor necrosis factor by its soluble receptors. J Exp Med 1992;175:323-9.
- Beutler B, van Huffel C. Unraveling function in the TNF ligand and receptor families. Science 1994;264:667-8.
- Leist M, Gantner F, Jilg S, Wendel A. Activation of the 55 kDa TNF receptor is necessary and sufficient for TNF-induced liver failure, hepatocyte apoptosis, and nitrite release. J Immunol 1995;154:1307-16.
- Punzon C, Alcaide A, Fresno M. In vitro anti-inflammatory activity of Phlebodium decumanum. Modulation of tumor necrosis factor and soluble TNF receptors. Int Immunopharmacol 2003;3:1293-9.
- Gambari R. Biospecific interaction analysis (BIA) as a tool for the design and development of gene transcription modifiers. Curr Med Chem 2001;1:277-91.
- Baker KN, Rendall MH, Patel A, Boyd P, Hoare M, Freedman RB, et al. Rapid monitoring of recombinant protein products: a comparison of different technologies. Trends Biotechnol 2002;20:149-56.
- Wilson WD. Analyzing biomolecular interactions. Science 2002;295:2103-5.
- Buckle PE, Davies RJ, Kinning T, Yeung D, Edwards PR, Pollard KD. The resonant mirror: a novel optical sensor for direct sensing of biomolecular interactions: Part II. Applications. Biosens Bioelectron 1993;8:355-68.
- Karlsson R, Michaelsson A, Mattsson L. Kinetic analysis of monoclonal antibody–antigen interactions with a new biosensor based analytical system. J Immunol Methods 1991;145:229-40.
- Dmitriev DA, Massino YS, Segal OL. Kinetic analysis of interactions between bispecific monoclonal antibodies and immobilized antigens using a resonant mirror biosensor. J Immunol Methods 2003;280:183-202.
- Bertucci C, Cimitan S, Menotti L. Optical biosensor analysis in studying herpes simplex virus glycoprotein D binding to target nectin1 receptor. J Pharm Biomed Anal 2003;32:697-706.
- Fang J, Wang DB, Chen CQ. Expression in BHK cells of two human TNF receptors and their interaction with hTNF-α. Acta Biochim Biophys Sin 1999;31:25-30.
- Carmen P, Antonio A, Manuel F. In vitro anti-inflammatory activity of Phlebodium decumanum. Modulation of tumor necrosis factor and soluble TNF receptors. Inter Immunopharm 2003;3:1293-9.
- Le Y. Clinical implication of soluble tumor necrosis factor receptors of infection diseases. Clin Med J Chin 2004;11:184-5.
- Klaus P. Biological functions of tumor necrosis factor cytokines and their receptors. Cytokine Growth Factor Rev 2003;14:185-91.
- Monika H, Thomas P, Janet MM. Diurnal and sleep-wake dependent variations of soluble TNF- and IL-2 receptors in healthy volunteers. Brain Behavior Immunity 2004;18:361-7.
- Jiang YR, Yin HJ, Chen KJ. Current status of research on paeoniae radix 801. Chin J Integ Tradit West Med 2004;24:760-3.