l-Stepholidine increases the frequency of sEPSC via the activation of D1 dopamine signaling pathway in rat prelimbic cortical neurons1
Introduction
l-Stepholidine (SPD), isolated from the Chinese herb Stephania, is a tetrahydroprotoberberine alkaloid. Previous studies in neuropharmacology, neurochemistry, electro-physiology, and behavioral experiments have demonstrated that SPD not only acts as a partial D1 agonist[1–5], but also as a full D2 antagonist[2,5,6]. SPD is the first known drug to possess the dual properties toward the dopamine (DA) receptor. Clinical trials have implicated that SPD is effective in the treatment of both positive and negative syndromes in schizophrenia[7]. Furthermore, SPD was also found to enhance the antipsychiatry effectiveness of other drugs and reduce tardive dyskinesia[8]. However, the mechanism of SPD’s anti-schizophrenia has not been explored.
The prelimbic cortex (PL) is well known as a critical structure in cognitive function. It is also believed that functional abnormalities in PL are associated with many neuropsychiatric disorders and symptoms including negative symptoms and cognitive impairment in schizophrenia[9–12]. The functional activity of the PL is mainly mediated by the intrinsic neurons and their interactions with other brain regions. The dopaminergic project from the ventral tegmental area (VTA) to the PL pyramidal cells located in layers V–VI is of particular importance for modulating PL function[13–19]. Particularly layers V contains a high density of D1 receptors[20]. Furthermore, many studies documented the importance of PL DA transmission via D1 receptors for optimal PL function[21,22]. D1 receptor stimulation is generally believed to promote N-methyl-D-aspartate (NMDA) receptor function on the excitable neurons via the medium spiny neuronal transmission, further supporting the hypothesis that DA transmission at D1 receptors in the PL is involved in the cognitive impairment and the negative symptoms of schizophrenia[12,19,23,24].
Anatomical studies have shown that the dendritic and somatic regions of PL pyramidal neurons receive synaptic inputs from both glutamatergic terminals arising from cortical and subcortical sources and dopaminergic afferents from VTA[25–29]. Furthermore, ultrastructural studies demonstrate that dopaminergic and glutamatergic axon terminals are in direct apposition to each other on the same postsynaptic pyramidal neuron in the PL which forms so-called “synaptic triads”, suggesting that both presynaptic and postsynaptic inputs participate in the modulation of synaptic transmission[30–32]. SPD is found to potently enhance the amplitude of NMDA-mediated currents in PL pyramidal neurons in brain slices via a postsynaptic mechanism[12]. However, the potential role of presynaptic regulation in mediating the effect of SPD is unknown and of interest since it has reported that DA can act on presynaptic D1 receptors and increase the frequency of sEPSC in PL pyramidal neurons in brain slices[33].
The present work was designed to study the effect and signaling mechanism for the SPD-modulated frequency of sEPSC in PL pyramidal neurons. The results indicate that presynaptic mechanism plays a critical role in the SPD-elicited effect on the frequency of sEPSC in PL pyramidal cells via D1 receptors.
Materials and methods
Preparation of prelimbic cortical slices Sprague-Dawley rats (Shanghai Experimental Animal Center, Chinese Academy of Sciences, Shanghai, China), weighing 30–50 g, were housed under standard laboratory conditions with constant temperature (22–23 °C) and humidity (50%–60%). All animal experiments were conducted in compliance with the Guide for the Care and Use of Laboratory Animals (National Research Council, 1996). Prelimbic cortical slices were prepared according to procedures described previously[33]. The rats were anesthetized with chloral hydrate (400 mg/kg, ip). Following decapitation, the brain was quickly removed and submerged in ice-cold perfusive medium containing 130 mmol/L NaCl, 5 mmol/L KCl, 2 mmol/L CaCl2, 2 mmol/L MgSO4, 1.25 mmol/L NaH2PO4, 26 mmol/L NaHCO3, 10 mmol/L glucose, 10 mmol/L sucrose, and saturated with 95% O2 and 5% CO2. The PL was removed and placed on a layer of moistened filter paper glued to the cutting stage of automatic oscillating tissue slicer (OTS-4000, Electron Microscopy Sciences, Fort Washington, PA, USA). Serial coronal slices (380 µm) were cut and transferred to an incubating chamber (28–30 °C) for at least 1 h before recording.
Visualization of pyramidal cells To visualize the cell, the slice was placed in a recording chamber and viewed with a fixed stage, upright microscope (BX51WI, Olympus, Tokyo, Japan). To increase the clarity of the cell, infrared light was used to illuminate the slice. The resultant infrared differential interference contrast (DIC) images were visualized on a black–white TV monitor through the use of a light sensitive charge coupled device (CCD) camera. Recordings were made from pyramidal cells located in layers V–VI. They were identified by their pyramidal shape, large soma, and presence of apical dendrites (Figure 1).
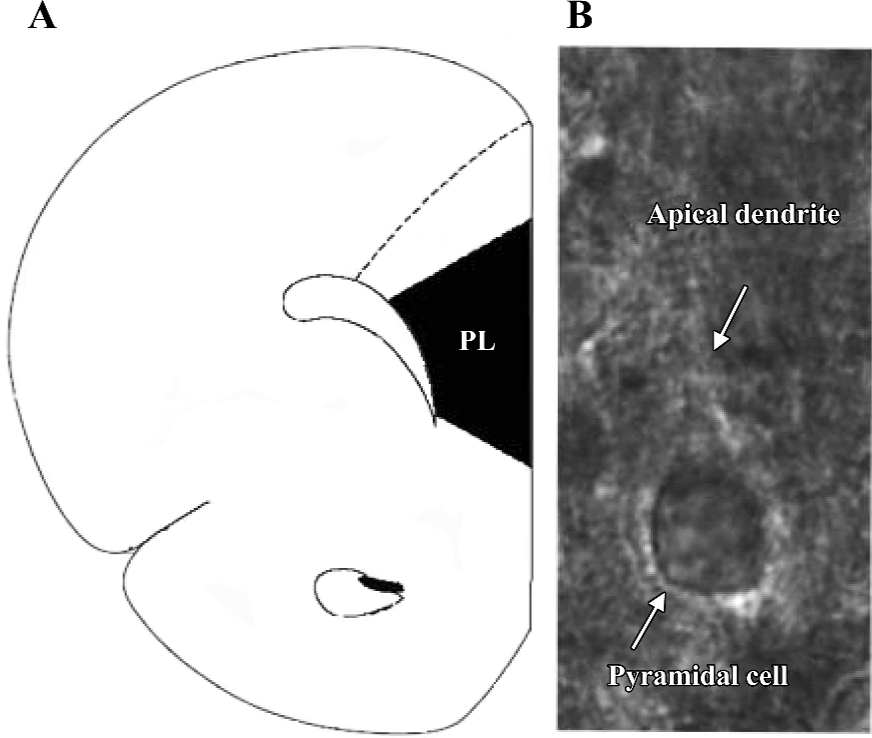
Whole-cell recording The slice was continuously perfused with the above perfusive medium and saturated with 95% O2 and 5% CO2. Electrodes (4–6 ΜΩ), pulled from glass capillaries with a Sutter micropipette puller (P-97, Novato, CA, USA), were filled with a solution (pH 7.25) containing 140 mmol/L K-gluconate, 0.1 mmol/L CaCl2, 2 mmol/L MgCl2, 1 mmol/L ethylene glycol tetraacetic acid (EGTA), 2 mmol/L ATP·K2, 0.1 mmol/L GTP·Na3, and 10 mmol/L N-(2-hydroxyethyl) piperazine-N’-(2-ethanesulfonic acid) (HEPES). Voltage and current signals were recorded with an Axon patch 700A amplifier (Union City, CA, USA) connected to a Digidata 1322A interface (Union City, CA, USA). The data were digitized and stored on disks using Clampfit (version 8.2.0.228, Axon, USA). Resting membrane potential and action potential were recorded under the current clamp mode. The recording pyramidal cell had a resting membrane potential less than -50 mV, an action potential amplitude greater than 80 mV, and no spontaneous action potentials. sEPSC, which had variable amplitude with a fast rising phase and a slower decay, were recorded at a holding potential of -70 mV (Figure 2A). These currents were blocked by an α-amino-3-hydroxy-5-methylisoxazole-4-propionic acid (AMPA)/kainite glutamate receptor antagonist 2,3-dihydroxy-6-nitro-7-sulfamoyl-benzo(f)quinoxaline (NBQX, 10 µmol/L). The series resistance was monitored by measuring the instantaneous current in response to a 5 mV voltage step command. Series resistance compensation was not used, and cells whose series resistance changed more than 15% were discarded.
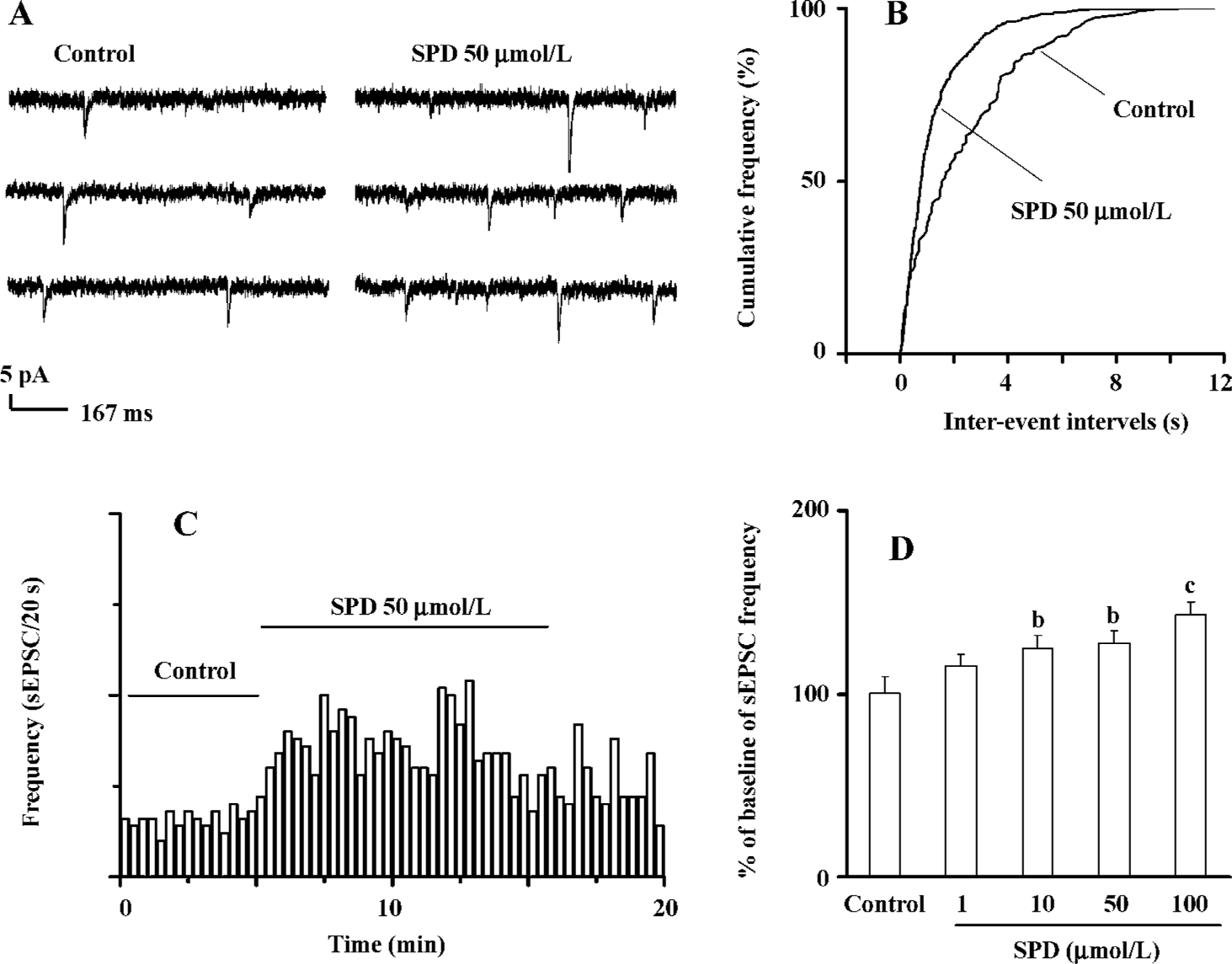
Offline data analysis Offline data analysis was performed using the Mini Analysis Program 5.0 (Synaptosoft, Fort Lee, NJ, USA) and Origin 7.0 (OriginLab Corporation, Northampton, MA, USA). The record of the sEPSC was shown with high resolution, and events that did not show a typical sEPSC waveform were rejected. The frequency and the inter-event intervals of sEPSC were measured. The effects of drugs were compared before and after drug injection with ANCOVA. The covariate was the baseline value prior to the drug injection. Statistical significance of distribution was made with the Kolmogorov-Smirnov (K-S) test. All numerical data were expressed as mean±SEM. In all cases, n refers to the number of cells studied.
Drugs SPD (Shanghai Institute of Materia Medica, Chinese Academy of Sciences, China) was dissolved in 0.1 mmol/L H2SO4, then diluted and neutralized with 0.1 mmol/L NaOH (pH 5.0). NBQX, K-gluconate, ATP·K2, GTP·Na3, SCH23390, N-(2-[p-bromocinnamylamino]-ethyl)-5-isoquinolinesulfonamide hydrochloride (H-89), SKF38393, sulpiride, and chelerythrine were purchased from Sigma (St Louis, MO, USA). All drugs were applied through bath perfusion.
Results
SPD increases frequency of sEPSC In PL pyramidal cells, bath application of SPD (50 µmol/L) resulted in an apparent increase in sEPSC frequency. A typical current trace is shown at Figure 2A. A plotting cumulative distribution of sEPSC intervals with the K-S statistical analysis indicated that SPD induced a clear shift towards shorter intervals in the cumulative curve (Figure 2B). On average, sEPSC frequency significantly increased after SPD (P<0.05, n=13 cells, Figure 2C). A SPD-elicited increase in sEPSC frequency was concentration dependent (n=5 cells, Figure 2D). A significant increase in the sEPSC (125.2%±6.8%, P<0.05) at 10 µmol/L was already observed; it reached its maximum at 100 µmol/L (P<0.01).
SPD-induced increase in sEPSC frequency is mediated by the D1 receptor The above results showed that SPD produced an increase of sEPSC frequency. We then determined the potential mechanism involved in the action of SPD. The application of the selective D1 receptor antagonist SCH23390 (10 µmol/L) significantly blunted the SPD-induced increase in sEPSC frequency (Figure 3A, 3B). The stimulation on sEPSC frequency was recovered while SCH23390 was washed out. This was further supported by data that the D1 partial agonist SKF38393 (10 µmol/L) mimicked the increase of SPD on the frequency of sEPSC (P<0.05, Figure 3E,F), while the D2/3 receptor antagonist sulpiride (10 µmol/L) elicited no effects on sEPSC frequency (n=6 cells, Figure 3C,D). Thus, it is clear that the D1 receptor, not the D2/3 receptor, mediated the effect of SPD on sEPSC frequency.
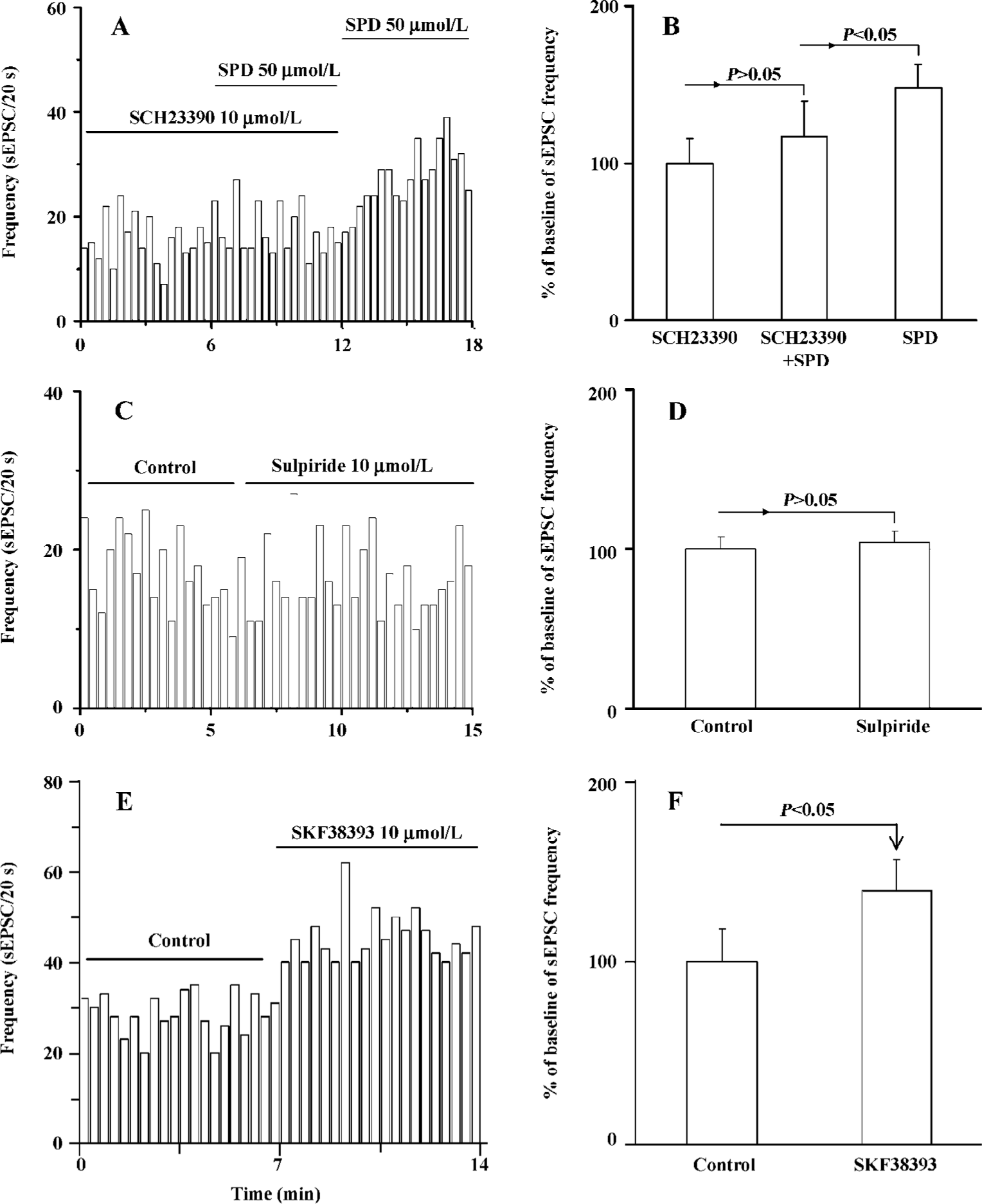
Both PKA and PKC are involved in SPD-induced increase in the frequency of sEPSC It is known that D1 receptor stimulation induces the activation of protein kinase A (PKA) and protein kinase C (PKC). We determined which intracellular pathway was involved in the SPD-altered frequency of sEPSC. As shown in Figure 4, when the specific PKA antagonist H-89 (10 µmol/L) was applied prior to or during SPD incubation, the SPD-elicited increase in the frequency of sEPSC was abolished. This was also true when the PKC inhibitor chelerythrine (2.5 µmol/L) was employed (Figure 4C, D). The results therefore implicated that both PKA and PKC were involved in the regulatory effect of SPD on sEPSC frequency.
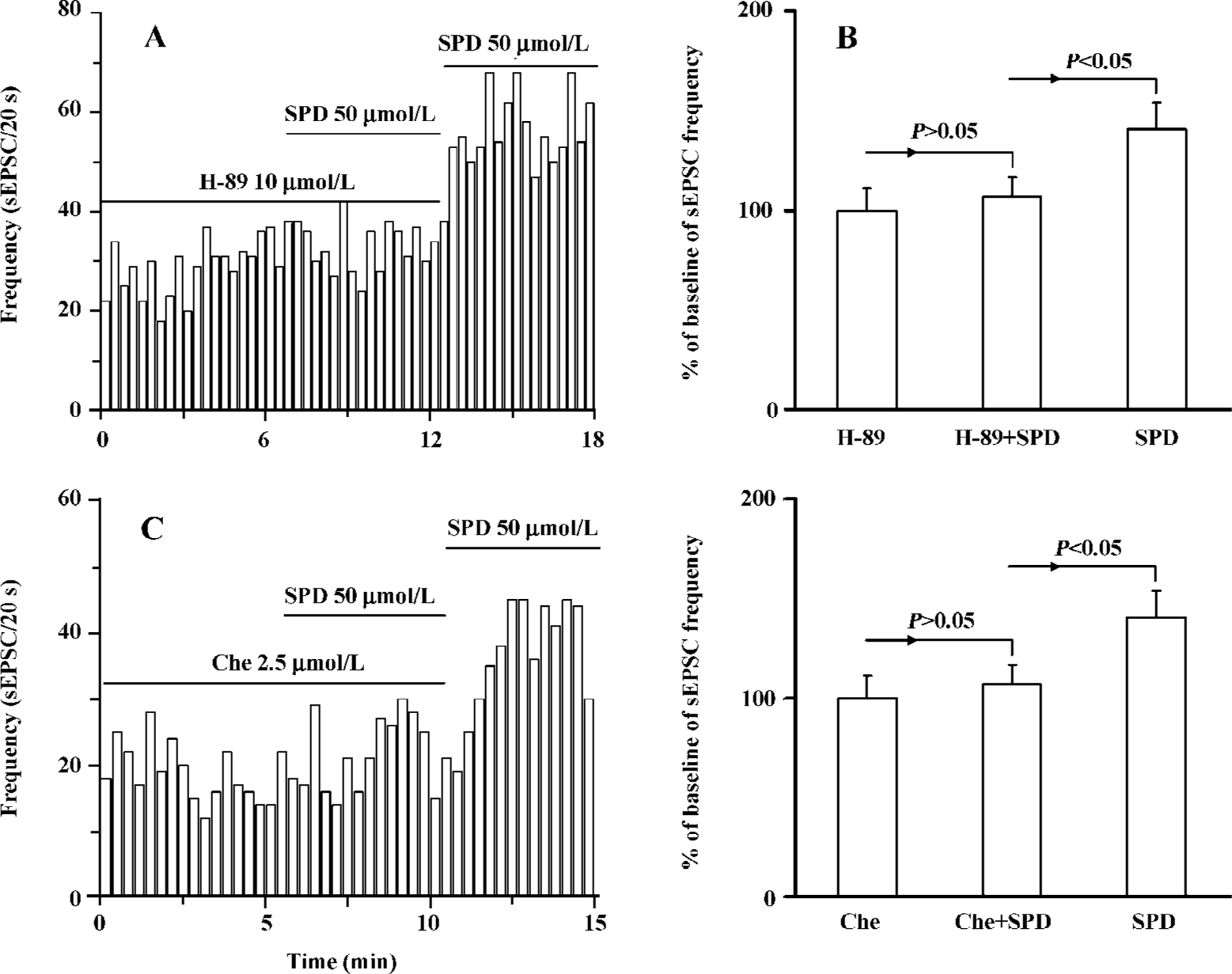
Discussion
The present study demonstrated that SPD significantly increased the frequency of sEPSC in a concentration-dependent manner. Selective D1 receptor antagonist SCH23390 blocked SPD-mediated effects, whereas D1 agonist SKF38393, but not the D2/3 antagonist sulpiride mimicked a SPD-mediated increase in the frequency of sEPSC. Moreover, both PKA inhibitor H-89 and PKC inhibitor chelerythrine attenuated the effect of SPD on sEPSC.
Previous studies from our laboratory and others have shown that SPD displays high affinity to both D1- and D2-like receptors. It is clear now that SPD possesses a dual property towards DA receptors. It acts as an agonist on the D1 receptor and functions as an antagonist on the D2/3 receptor[5,34-40]. Our data demonstrated that enhancement of sEPSC frequency in the PL pyramidal cells by SPD was mediated by the D1 receptor, whereas D2/3 receptors appeared not to associate with the action.
It is known that sEPSC are mainly resulted from the action potential-dependent glutamate release and thereby the increase in sEPSC frequency could be due to the activation of some neurotransmitter receptors, such as the D1 receptor, that result in synaptic transmission from a presynaptic action[33,41-43]. In agreement with previous finding that D1 receptors could modulate presynaptic glutamate release[44,45], the present study showed that SPD increased sEPSC frequency via D1 receptors. It therefore appeared that the underlying mechanism for the SPD-mediated increase in sEPSC frequency is associated with the drug-induced presynaptic release of glutamate, and subsequently, the modulation of the synaptic inputs to PL pyramidal cells.
Both PKA and PKC are known to be the important signaling molecules that transduce D1-like receptor signals[46,47]. A previous study demonstrated that DA stimulated the frequency of sEPSC through PKA and PKC in rat PL pyramidal cells via the presynaptic D1 receptor[33]. Present data also indicate that PKA and PKC play an important role in SPD-mediated modulation on sEPSC frequency. Therefore, it appears that both DA and SPD share common signaling pathways via the activation of the D1 receptor in the modulation of sEPSC frequency.
More importantly, the present data provides novel evidence for the functional interaction between the D1 and NMDA receptors in neurons. It is known that the interaction between the D1 and NMDA receptors plays a critical functional role in the frontal cortex. It is interesting to note that the suggested alterations of the D1–NMDA receptor interaction in the PL are important pathological mechanisms underlying the neurochemical imbalance in schizo-phrenia[12,19]. Both the D1 and NMDA receptors in the frontal cortex play a critical role in synaptic plasticity, memory, and cognition. Dysfunction of NMDA and D1 receptor is believed to associate with the negative symptoms and cognitive impairment in schizophrenia[48-50]. Our results show that SPD increases the frequency of sEPSC via the activation of D1 receptors located in the PL V–VI layers, presumably through enhancing the presynaptic glutamate release. Considering the advantageous benefits of SPD on negative symptoms of schizophrenic patients[7], the present study provides a potential molecular mechanism for the treatment of SPD on negative symptoms of schizophrenia. Furthermore, unique pharmacological properties of SPD (the D1 agonist in the PL and the D2/D3 antagonist in the subcortex) lead us to believe that SPD is a promising candidate as a novel antipsychotic agent.
References
- Dong ZJ, Chen LJ, Jin GZ, Creese I. Gtp regulation of (-)-stepholidine binding to R(h) of D1 dopamine receptors in calf striatum. Biochem Pharmacol 1997;54:227-32.
- Jin GZ, Zhu ZT, Fu Y. (-)-stepholidine: A potential novel antipsychotic drug with dual D1 receptor agonist and D2 receptor antagonist actions. Trends Pharmacol Sci 2002;23:4-7.
- Shi WX, Chen Y, Jin GZ. Effect of l-stepholidine on rotational behavior in rats. Acta Pharmacol Sin 1984;5:222-5.
- Xu SX, Yu LP, Han YR, Chen Y, Jin GZ. Effects of tetrahydro-protoberberines on dopamine receptor subtypes in brain. Acta Pharmacol Sin 1989;10:104-10.
- Zou LL, Liu J, Jin GZ. Involvement of receptor reserve in D1 agonistic action of (-)-stepholidine in lesioned rats. Biochem Pharmacol 1997;54:233-40.
- Dong ZJ, Guo X, Chen LJ, Han YF, Jin GZ. Dual actions of (-)-stepholidine on the dopamine receptor-mediated adenylate cyclase activity in rat corpus striatum. Life Sci 1997;61:465-72.
- Wu DC, Xing XZ, Wang WA, Xie H, Wang YH, Wang XY, et al. A double-blind comparison trial of l-stepholidine and perphenazine in treatmeat of schizophrenia. Chin J New Drugs Clin Remedies 2003;22:155-9.
- Cai N. A controlled study on the treatment of tardive dyskinesia using l-stepholidine. Zhonghua Shen Jing Jing Shen Ke Za Zhi 1988;21:281-3.
- Goldman-Rakic PS. The cortical dopamine system: Role in memory and cognition. Adv Pharmacol 1998;42:707-11.
- Goldman-Rakic PS, Castner SA, Svensson TH, Siever LJ, Williams GV. Targeting the dopamine D1 receptor in schizophrenia: Insights for cognitive dysfunction. Psychopharmacology (Berl) 2004;174:3-16.
- Seamans JK, Yang CR. The principal features and mechanisms of dopamine modulation in the prefrontal cortex. Prog Neurobiol 2004;74:1-58.
- Yang CR, Chen L. Targeting prefrontal cortical dopamine D1 and n-methyl-d-aspartate receptor interactions in schizophrenia treatment. Neuroscientist 2005;11:452-70.
- Brozoski TJ, Brown RM, Rosvold HE, Goldman PS. Cognitive deficit caused by regional depletion of dopamine in prefrontal cortex of rhesus monkey. Science 1979;205:929-32.
- Jentsch JD, Roth RH, Taylor JR. Role for dopamine in the behavioral functions of the prefrontal corticostriatal system: Implications for mental disorders and psychotropic drug action. Prog Brain Res 2000;126:433-53.
- Lindstrom LH, Gefvert O, Hagberg G, Lundberg T, Bergstrom M, Langstrom B, et al. Increased dopamine synthesis rate in medial prefrontal cortex and striatum in schizophrenia indicated by l-(beta-11c) dopa and pet. Biol Psychiatry 1999;46:681-8.
- Murphy BL, Arnsten AF, Goldman-Rakic PS, Roth RH. Increased dopamine turnover in the prefrontal cortex impairs spatial working memory performance in rats and monkeys. Proc Natl Acad Sci USA 1996;93:1325-9.
- Tzschentke TM. Pharmacology and behavioral pharmacology of the mesocortical dopamine system. Prog Neurobiol 2001;63:241-320.
- Winn P. Schizophrenia research moves to the prefrontal cortex. Trends Neurosci 1994;17:265-8.
- Abi-Dargham A, Laruelle M. Mechanisms of action of second generation antipsychotic drugs in schizophrenia: Insights from brain imaging studies. Eur Psychiatry 2005;20:15-27.
- Goldman-Rakic PS, Lidow MS, Gallager DW. Overlap of dopaminergic, adrenergic, and serotoninergic receptors and complementarity of their subtypes in primate prefrontal cortex. J Neurosci 1990;10:2125-38.
- Goldman-Rakic PS, Muly EC 3rd, Williams GV. D1 receptors in prefrontal cells and circuits. Brain Res Rev 2000;31:295-301.
- Sawaguchi T, Goldman-Rakic PS. D1 dopamine receptors in prefrontal cortex: Involvement in working memory. Science 1991;251:947-50.
- Davis KL, Kahn RS, Ko G, Davidson M. Dopamine in schizo-phrenia: A review and reconceptualization. Am J Psychiatry 1991;148:1474-86.
- Weinberger DR. Implications of normal brain development for the pathogenesis of schizophrenia. Arch Gen Psychiatry 1987;44:660-9.
- Berger B, Thierry AM, Tassin JP, Moyne MA. Dopaminergic innervation of the rat prefrontal cortex: A fluorescence histochemical study. Brain Res 1976;106:133-45.
- Conde F, Audinat E, Maire-Lepoivre E, Crepel F. Afferent connections of the medial frontal cortex of the rat. A study using retrograde transport of fluorescent dyes. I. Thalamic afferents. Brain Res Bull 1990;24:341-54.
- Herkenham M. Laminar organization of thalamic projections to the rat neocortex. Science 1980;207:532-5.
- Penit-Soria J, Audinat E, Crepel F. Excitation of rat prefrontal cortical neurons by dopamine: An in vitro electrophysiological study. Brain Res 1987;425:263-74.
- Ray JP, Price JL. The organization of the thalamocortical connections of the mediodorsal thalamic nucleus in the rat, related to the ventral forebrain-prefrontal cortex topography. J Comp Neurol 1992;323:167-97.
- Carr DB, Sesack SR. Hippocampal afferents to the rat prefrontal cortex: Synaptic targets and relation to dopamine terminals. J Comp Neurol 1996;369:1-15.
- Goldman-Rakic PS, Leranth C, Williams SM, Mons N, Geffard M. Dopamine synaptic complex with pyramidal neurons in primate cerebral cortex. Proc Natl Acad Sci USA 1989;86:9015-19.
- Smiley JF, Goldman-Rakic PS. Heterogeneous targets of dopamine synapses in monkey prefrontal cortex demonstrated by serial section electron microscopy: A laminar analysis using the silver-enhanced diaminobenzidine sulfide (SEDS) immunolabeling technique. Cereb Cortex 1993;3:223-38.
- Wang Z, Feng XQ, Zheng P. Activation of presynaptic D1 dopamine receptors by dopamine increases the frequency of spontaneous excitatory postsynaptic currents through protein kinase A and protein kinase C in pyramidal cells of rat prelimbic cortex. Neuroscience 2002;112:499-508.
- Liu J, Guo X, Wang BC, Jin GZ. Increased phosphorylation of DARPP-32 by D1 agonistic action of l-stepholidine in the 6-OHDA-lesioned rat striatum. Acta Physiol Sin 1999;51:65-72.
- Sun BC, Zhang XX, Jin GZ. (-)-Stepholidine acts as a D1 partial agonist on firing activity of substantia nigra pars reticulata neurons in 6-hydroxydopamine-lesioned rats. Life Sci 1996;59:299-306.
- Zhang XX, Liu J, Fu Y, Hu GY, Jin GZ. Action sites of rotation and unit firing induced by l-stepholidine and DA agonists in basal ganglia of 6-OHDA-lesioned rats. Acta Pharmacol Sin 1999;20:979-86.
- Zhu ZT, Fu Y, Hu GY, Jin GZ. Electrophysiological study on biphasic firing activity elicited by D1 agonistic-D2 antagonistic action of (-)-stepholidine in nucleus accumbens. Acta Physiol Sin 2000;52:123-30.
- Zhu ZT, Fu Y, Hu GY, Jin GZ. Modulation of medical prefrontal cortical D1 receptors on the excitatory firing activity of nucleus accumbens neurons elicited by (-)-stepholidine. Life Sci 2000;67:1265-74.
- Zhu ZT, Wu WR, Fu Y, Jin GZ. l-stepholidine facilitates inhibition of mPFC DA receptors on subcortical NAc DA release. Acta Pharmacol Sin 2000;21:663-7.
- He Y, Yu LP, Jin GZ. Altered rotation behavior of sensitized rats to effect of l-stepholidine: Implication of dopamine D3R antagonist. Pharmacol Biochem Behav 2007. in press.
- Feng XQ, Dong Y, Fu YM, Zhu YH, Sun JL, Zheng P, et al. Progesterone inhibition of dopamine-induced increase in frequency of spontaneous excitatory postsynaptic currents in rat prelimbic cortical neurons. Neuropharmacology 2004;46:211-22.
- Parfitt KD, Madison DV. Phorbol esters enhance synaptic transmission by a presynaptic, calcium-dependent mechanism in rat hippocampus. J Physiol 1993;471:245-68.
- Wang Z, Zheng P. Characterization of spontaneous excitatory synaptic currents in pyramidal cells of rat prelimbic cortex. Brain Res 2001;901:303-13.
- Bouron A, Reuter H. The D1 dopamine receptor agonist SKF-38393 stimulates the release of glutamate in the hippocampus. Neuroscience 1999;94:1063-70.
- Hu XT, White FJ. Dopamine enhances glutamate-induced excitation of rat striatal neurons by cooperative activation of D1 and D2 class receptors. Neurosci Lett 1997;224:61-5.
- Kebabian JW, Petzold GL, Greengard P. Dopamine-sensitive adenylate cyclase in caudate nucleus of rat brain, and its similarity to the “Dopamine receptor”. Proc Natl Acad Sci USA 1972;69:2145-9.
- Hussain T, Lokhandwala MF. Renal dopamine receptor function in hypertension. Hypertension 1998;32:187-97.
- Kretschmer BD. Modulation of the mesolimbic dopamine system by glutamate: Role of nmda receptors. J Neurochem 1999;73:839-48.
- Olney JW, Newcomer JW, Farber NB. NMDA receptor hypofunction model of schizophrenia. J Psychiatr Res 1999;33:523-33.
- Svensson TH. Dysfunctional brain dopamine systems induced by psychotomimetic NMDA-receptor antagonists and the effects of antipsychotic drugs. Brain Res Rev 2000;31:320-9.