Expression of human insulin gene wrapped with chitosan nanoparticles in NIH3T3 cells and diabetic rats
Introduction
Type 1 diabetes mellitus is due to the autoimmune-mediated destruction of pancreatic B cells, which results in absolute insulin deficiency[1]. Hyperglycemia can cause long-term clinical problems, including renal failure, retinopathy, neuropathy, and heart disease[2]. Although intensive exogenous insulin therapy can delay or prevent the onset of chronic complications, it is rather cumbersome and can cause hypoglycemia, which could be life threatening. However, the development of gene therapy has also generated much hope and excitement for a possible cure for diabetes since the insulin gene was first cloned and expressed in cultured cells in the late 1970s[3].
One factor critical to successful gene therapy is the development of efficient delivery systems. Although advances in gene transfer technology, including viral and non-viral vectors have been made, an ideal vector system has not yet been constructed[4]. Although viral vectors can introduce exogenous genes into cells precisely and effectively, it can easily cause immune reactions because of the existence of antivirotic immune system. Due to growing concerns over the toxicity and immunogenicity of viral DNA delivery systems, DNA delivery via improved viral routes has become more desirable[5].
A perfect vector should also be biocompatible, efficient, and modular so that it is tunable to various applications in both research and clinical settings[6]. Taken into account this point, we selected chitosan nanoparticles, a non-viral vector, in the present study. Non-viral vectors include liposomes[7–9], composites[10], microspheres[11], and nanoparticles[12,13], but the cytotoxicity of the liposomes limits its application in vivo. Owing to its loose constitution, the constancy of composites is poor. The diameter of microspheres is greater than that of the nanoparticles. Therefore, chitosan nanoparticles are a promising, non-viral vector comparatively.
In conventional insulin gene therapy, the exogenous insulin gene is usually transferred by caudal vein, portal vein, intraperitoneal, or intramuscular injection. They are invasive methods and can result in infections or death. The wounds and infections can also influence the blood glucose level and the effect of gene therapy in diabetic rats significantly. The target cells in these methods mainly focus on hepatic and skeletal muscle cells, but hepatic and skeletal muscle cells are not endocrinal cells, and are quite different from β cells; they usually need to be genetically modified in response to glucose and processing from pro-insulin to insulin. There is a considerable amount of endocrinal cells in gastrointestinal tracts, which are considered ideal target cells in the gene therapy of diabetes[14,15]. Considering these facts, we attempted to transfer the exogenous human insulin gene by gastrointestinal tracts. The transfer of the human insulin gene to diabetic rats by gastrointestinal tracts is very safe, painless, and can effectively avoid infections.
In the present study, we constructed an expression plasmid pCMV.Ins expressing the human insulin gene. We then wrapped pCMV.Ins with chitosan nanoparticles, a non-viral vector, which was transfected to NIH3T3 cells and diabetic rats to evaluate the efficiency of chitosan nanoparticles as a vector and novel route for the transfection of the human insulin gene in diabetic rats. At present, there is no report for the transfection of the human insulin gene to diabetic rats through gastrointestinal tracts. There is also no report about the application of chitosan as a vector in gene therapy for type 1 diabetes mellitus.
Materials and methods
Construction of the expression plasmid The cDNA sequences of the human insulin gene were cut from the PBAT16, hInsG1.M2 (presented by Dr Michael GERMAN, Department of Hormone Research Institute, University of San Francisco, CA, USA) using the Bg1II/NotI enzyme. It was inserted in the expression vector pCMV.eGFP at the cohesive end. Then the recons, called pCMV.Ins, were transformed into Escherichia coli Top10F strains (Invitrogen, Carlsbad, CA, USA) and screened. Plasmids that were isolated using a large-scale alkaline lysis procedure were purified.
Identification of the plasmid pCMV.Ins was identified by PCR. The primers used for human insulin specifically were 5′-ATCACTGTCCTTCTGCCA-3′ (forward) and 5′-GGGTGTGTAGAAGAAGCC-3′ (reverse). The PCR products were analyzed by 1.5% agarose gel electrophoresis, and the expected amplification length was 173 bp. The DNA sequencing of the pCMV.Ins plasmid was performed in the Shanghai Sangon Sequencing Center (Shanghai, China).
Preparation of the chitosan–DNA nanoparticles Chitosan (0.1 g) was dissolved in 100 mL acetic acid, and the pH was adjusted to 5.5 with sodium hydrate. The solution was stored at 4 °C after being degermed by micropore film, of which the aperture was 0.22 μm. Before it was used, the chitosan solution was diluted with ddH2O until the concentration was 0.02%. In total, 100 μL DNA solution, (200 μg/mL concentration) was added to the 100 μL sodium sulfate solution (25 mmol/L concentration). After the chitosan and DNA solutions were heated to 55 °C in an aqueous bath for 15 min, respectively, they were immediately mixed together. Then they would be convoluted for 1 min. The volume of the reaction system did not excess 500 μL, as previously suggested[16], and the final concentration of the chitosan–DNA nanoparticles was 100 μg/mL.
Cell line and cell culture The NIH3T3 cell line was obtained from the China Center for Type Culture Collection (Wuhan University, Wuhan, China). The cells were cultured in Dulbecco’s modified Eagle’s medium (DMEM; Sigma, St Louis, MO, USA) and 10% fetal bovine serum GibcoBRL, Gaithersburg, MD, USA) in a 5% humidified CO2 atmosphere at 37 ºC. The cells were transfected by pCMV.eGFP and pCMV.Ins wrapped with chitosan nanoparticles (chitosan–pCMV.Ins), respectively. The cells were grown in DMEM, containing 700 μg/mL G418, at 72 h after transfection. The cell clones were selected and continued to grow in 400 μg/mL G418 (GibcoBRL, Gaithersburg, MD, USA) medium until the d 24.
Analysis of immunohistochemistry in the transfected cells Human insulin in the transfected cells was detected by immunohistochemistry using a specific antibody against human insulin (Sigma, USA). The cells were collected by a brief trypsinization, smeared, and stained using normal SP immunohistochemical method. The color was observed with 3,3′-diaminobenzidine (DAB) reagent and stained again with hematein. Hematein-stained cells were examined under a light microscope and photographed. Cells that were positive for human insulin expression had brown plasma. Five fields from 6 different wells were randomly selected from each group, and the number of positive cells was counted manually. The ratio of positive cells to total cells was calculated.
Detection of human insulin in transfected cells Human insulin in the culture medium of cells transfected by pCMV.eGFP and chitosan–pCMV.Ins was measured. Human insulin was determined every second day until d 24 with a commercial human insulin radioimmunoassay (RIA) kit from the China Institute of Atomic Energy (Beijing, China).
Preparation of the animal model Experiments were carried out on 45 8-week-old male Wister rats (180–190 g). After an overnight fast, the rats were given a single intraperitoneal injection of 60 mg/kg streptozotocin (STZ; Upjohn, Kalamazoo, MI, USA) in 0.01 mol/L citrate buffer. Forty rats with fasting blood glucose levels >16.7 mmol/L were used in the study. The rats were randomly divided into 4 groups. A single 1 mL dose of chitosan–pCMV.Ins nanoparticles (containing 100 μg pCMV.Ins) was transfected to the diabetes rats through lavage and coloclysis, respectively. The 2 control groups were treated with chitosan and normal saline, respectively.
Detection of fasting blood glucose and plasma insulin in diabetic rats The fasting blood glucose and plasma insulin of diabetic rats were measured in all of the groups every morning after 8 h of fasting. The rats had access to food and water ad libitum for the remaining 16 h. The glucose levels of the blood samples obtained from the tail veins were determined by One Touch meters and test strips (Lifescan, USA) every morning. The plasma human insulin level was measured with a commercial human RIA kit from the China Institute of Atomic Energy.
RT-PCR analysis in diabetic rats RT–PCR was used to verify the expression of the human insulin gene in gastrointestinal tracts. Half the diabetic rats in each group were killed on d 5. Immediately after, the stomachs, intestines, and rectums of the treated and control animals were flash frozen in liquid nitrogen and then stored at –70 °C. Total RNA (5 μg), obtained by using TRIzol (GibcoBRL, USA) as per manufacturer’s instructions, was subjected to reverse transcription by using an oligo dT21 primer, recombinant RNAsin, and Avian Myeloblastosis Virus (AMV) reverse transcriptase (Promega, Madison, WI, USA). The AMV reverse transcriptase was inactivated at 95 °C, and the PCR reactions were performed by using a GeneAmp PCR system 9600 thermal cycler and Taq DNA polymerase (Perkin–Elmer, Norwalk, CT, USA). The cDNA mixture was allowed to react for 19 (GAPDH), or 22 (insulin) cycles. The primers used for human insulin were: 5′-ACCATGGCCCTGTGGATGCGC-3′ (forward), and 5′-CTAGTTGCAGTAGTTCTCCAG-3′ (reverse). The primers for GAPDH were: 5′-ACCACAGTCCATGCCATCAC-3′ (forward) and 5′-TCCACCACCCTGTTGCTGTA-3′ (reverse). Insulin primers were designed to amplify human insulin specifically. The primers for GAPDH were used in the control reactions. The RT–PCR products were analyzed by 1.5% agarose gel electrophoresis, and the expected amplification lengths were 336 and 451 bp, respectively.
Western blot analysis in diabetic rats On d 5 after the transfection, the stomachs, intestines, and rectums were harvested and resuspended in lysis buffer (1% Nonidet P-40, 50 mmol/L Tris-HCl, pH 7.4, 150 mmol/L NaCl, 200 U/mL aprotinin, and 1 mmol/L phenylmethanesulfonyl fluoride). The tissue lysates (50 mg protein) were separated by 12% polyacrylamide gel electrophoresis and blotted onto polyvinylidene difluoride membranes. Immunoblotting was performed with the antibody against human insulin (Sigma, USA). The molecular weight of the human insulin was 56 kDa.
Statistical analysis Data were expressed as mean±SD. The concentrations of blood glucose and plasma insulin were evaluated by Student’s t-test and one-way ANOVA, respectively (SPSS for Windows 11.5; SPSS, Chicago, IL, USA). Differences were considered significant when P-values were less than 0.05.
Results
Identification of recombinant plasmid pCMV.Ins The PCR products of recombinant plasmid pCMV.Ins and bacterial suspension were amplified. One specific band was obtained in lane 4 and lanes 5–7, respectively, corresponding to the expected size of 173 bp. No fragments in lanes 1 and 3 were amplified from pCMV.eGFP and the negative control (Figure 1). This result suggests that the human insulin gene was inserted into recombinant plasmid pCMV.Ins successfully. The sequencing results also revealed that the recombinant pCMV.Ins plasmid was successfully constructed. The number of sequencing report is LE142.
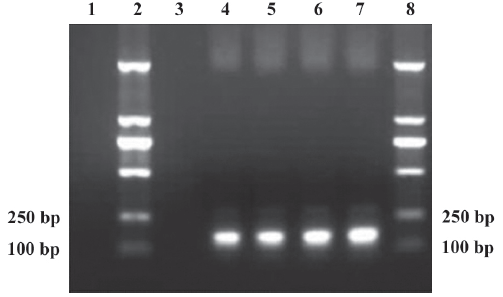
Expression of the human insulin gene in transfected cells Approximately 10% of NIH3T3 cells transfected by chitosan–pCMV.Ins expressed human insulin. The cells that were positive for human insulin expression had brown plasma (Figure 2A). There was no expression of human insulin in the NIH3T3 cells transfected by pCMV.eGFP. No changes were seen in the plasma with DAB staining in the negative cells, and nuclei in negative cells were stained blue (Figure 2B). These results suggested that human insulin was expressed by the exogenous chintosan–pCMV.Ins in NIH3T3 cells, but not by the endogenous insulin gene.
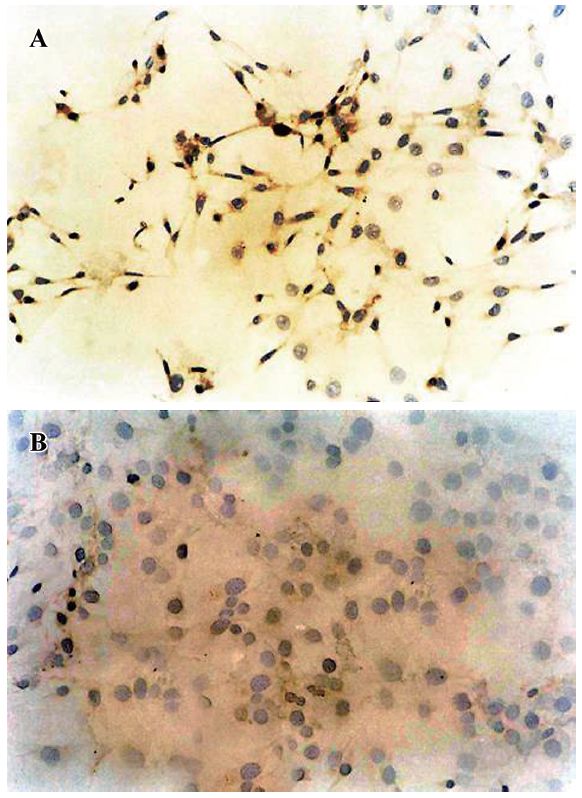
Level of insulin in the culture medium of transfected cells The levels of extracellular insulin in the cells transfected by chitosan–pCMV.Ins and pCMV.eGFP were 5.20±0.58 and 3.30±0.31 μIU/mL, respectively, on the d 24 (Table 1). The extracellular insulin levels in the chitosan–pCMV.Ins group increased significantly compared with those of the pCMV.eGFP group on d 24 (P<0.05). These results suggested that NIH3T3 cells transfected by chitosan–pCMV.Ins expressed human insulin successfully.

Full table
Change in fasting blood glucose in diabetic rats Fasting blood glucose was ameliorated. The fasting blood glucose in the lavage and coloclysis groups decreased from 22.31±1.38 to 5.63±0.48 mmol/L, and from 21.84±1.21 to 5.07±0.37 mmol/L after transfection on d 4, respectively. The lavage and coloclysis groups transfected with chitosan–DNA nanoparticles had significantly lower levels of fasting blood glucose (P<0.01) compared with the normal saline treatment from d 1–5 (Figure 3). The blood glucose levels of the chitosan group also decreased significantly, because chitosan ameliorated blood glucose. The blood glucose levels of the lavage and coloclysis groups were both significantly lower than those of the chitosan group (P<0.01). There were no differences in blood glucose levels at d 5 between the lavage and coloclysis groups (P=0.746).
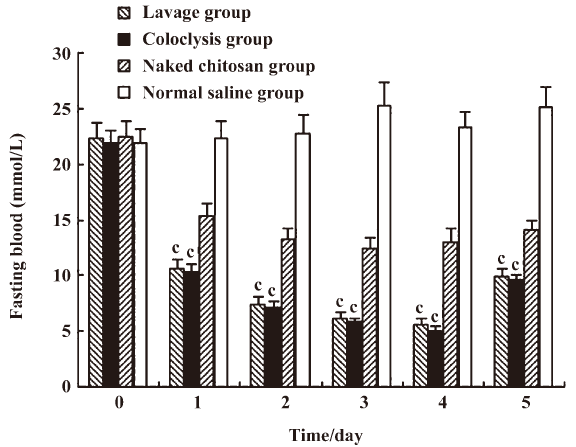
Change of plasma insulin in diabetic rats The levels of plasma insulin in the lavage and coloclysis groups increased from 14.01±1.24 to 32.26±1.81 μIU/mL, and from14.47±1.49 to 32.79±1.84 μIU/mL after transfection on d 4, respectively. The plasma insulin levels of the lavage, coloclysis, and chitosan groups (Figure 4) were higher than those of the normal saline group on d 1–5 (P<0.01). There were remarkable differences between the lavage and chitosan group (P<0.01), with little difference between the coloclysis and chitosan groups (P<0.01), and between the lavage and coloclysis groups (P=0.56). These results are consistent with the reduction of fasting blood glucose.
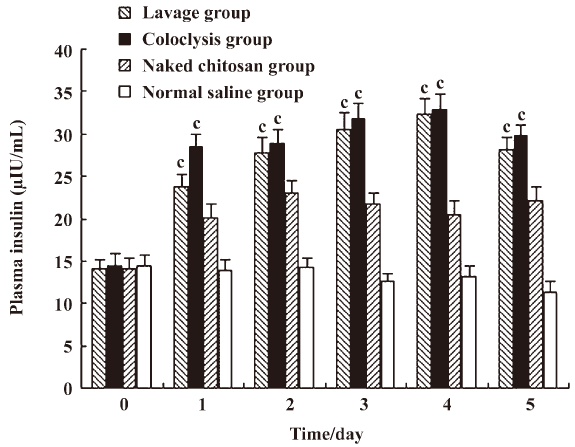
Verification of the expression of the human insulin gene in diabetic rats To analyze the expression of the human insulin gene, RT–PCR and Western blot analysis were carried out in the stomachs, intestines, and rectums of diabetic rats. The human insulin gene and human insulin were only detected in the intestines of diabetic rats. In the reactions using primers specific for GAPDH, RNA of the intestines were amplified, revealing a 451 bp fragment in all of the groups (Figure 5A). However, human insulin-specific primers produced a fragment corresponding to the expected size of 336 bp in lanes 1 and 2, only in the reactions containing RNA from the lavage and coloclysis groups, respectively (Figure 5B). No products were amplified from the cDNA of the chitosan (lane 3) and normal saline groups (lane 4).
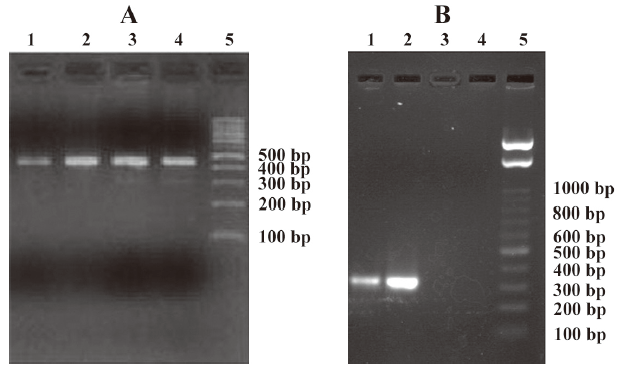
The molecular weight of human insulin was 56 kDa (Figure 6). One specific band was only obtained in the intestines of the lavage and coloclysis groups (lanes 1 and 2, respectively). No products were obtained in the chitosan group (lane 3) and normal saline group (lane 4). These results suggested that the detected mRNA and human insulin were expressed by the exogenous chitosan–pCMV.Ins, not by the endogenous insulin gene.
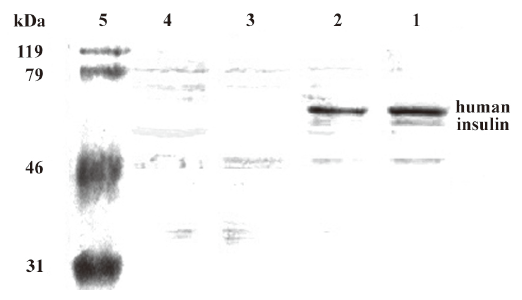
Discussion
Many attempts have been made in gene therapy for type 1 diabetes mellitus, including islet[17–19] and whole pancreas transplantation[20,21], the regeneration of β cells[22–24], and insulin gene therapy[25–27]. Whole organ transplants have been found to be sustainable and durable. Advances in islet transplantation procedures now mean that patients with the disease can be cured by transplantation of primary human islets of Langerhans. The major drawbacks in these strategies are the availability of donor islets, the invasiveness of the procedure, and high cost. Due to the limited number of donor islet cells available, researchers are looking for different sources of pancreatic islet progenitor or stem cells. A stem cell with proliferative ability may provide a valuable source of islet progenitor cells. Several studies have demonstrated that a progenitor/stem cell population can be expanded in vitro to generate large numbers of islet progenitor cells[28–30]. However, the efficient and directed differentiation of these cells to an endocrinal pancreatic lineage has been difficult to achieve.
Based on this, we attempted to apply insulin gene therapy in type 1 diabetic rats in the present study. Insulin gene therapy involves the introduction of a foreign gene into any cell type in the body, allowing it to produce insulin[31]. The gene(s) introduced could be the insulin gene itself, perhaps under the control of a tissue-specific promoter, allowing for the expression in a select non-β-cell type or a gene encoding a factor that in turn activates the insulin gene, thereby allowing for ectopic insulin production.
Our findings demonstrated that the human insulin gene wrapped by chitosan nanoparticles can be expressed efficiently in NIH3T3 cells and the gastrointestinal tracts of diabetes rats, indicating that chitosan nanoparticles are a non-viral vector with potential for gene expression. Chitosan nanoparticles[32] have the following benefits: good biocompatibility, no toxicity, and can be obtained economically. The transfection efficiency of chitosan can be regulated by changing its molecular weight, plasmid concentration, and the ratio of chitosan and plasmid. After the plasmid is embedded in chitosan, it can resist the degradation of the nuclei. It also exhibits antibacterial activity by inhibiting the bacterium metabolism. Köping–Höggård et al[33] found that aerosol delivery via formulation with chitosan oligomers resulted in improved lung distribution of pDNA polyplexes and a 6-fold increase in the efficiency of gene delivery in vivo over that seen with the commonly-used intratracheal instillation method.
In the present study, we transferred the human insulin gene to diabetic rats by gastrointestinal tracts. This is quite different from conventional insulin gene therapy. The exogenous insulin gene was transferred to diabetic rats by caudal vein, portal vein, intraperitoneal, or intramuscular injection in conventional insulin gene therapy. Koya et al[34] injected recombinant pancreatic duodenal homeobox-1 (rPdx1) into mice with STZ-induced diabetes by peritoneum. They found that the rPdx1 treatment of mice with STZ-induced diabetes promoted β-cell regeneration and liver cell reprogramming, leading to restoration of normoglycemia. These methods will injure diabetic rats and may lead to infections or death. The wounds and infections can also influence the blood glucose level and the effects of gene therapy in diabetic rats significantly. The human insulin gene transferred to diabetic rats by gastrointestinal tracts is very safe, painless, and can avoid infections effectively. In the present study, none of the diabetic rats was infected.
In insulin gene therapy, one of the key issues is the selection of carriers. Conventional viral vectors can introduce exogenous genes into cells precisely and effectively, but it can easily cause immune reactions because of the existence of the antivirotic immune system. More and more researchers are interested in non-viral vectors. Chitosan is an ideal and new non-viral vector and has drawn wide attention. We selected chitosan as the vector in this study, and the results demonstrated that chitosan was a safe and effective gene delivery vector.
In our study, we observed that the decrease of fasting blood glucose lasted for 5 d, which could be a result of the regeneration of gastrointestinal tract epithelial cells. We speculate that a single 1 mL dose of chitosan–DNA nanoparticles (containing 100 μg pCMV.Ins) may be cleared with the regeneration of gastrointestinal tract epithelial cells. As already known, the regeneration of gastrointestinal tract epithelial cells may require 3–5 day[35]. Our results also correspond with this point.
Further research will be undertaken to detect the cells that takes up the plasmid in vivo. We speculate that gut K cells may take up the expression plasmid. There are considerable gut K cells in the epithelium mucosa of gastrointestinal tracts that create PC2 and PC3 and secrete glucose-dependent insulinotropic polypeptides (GIP)[36]. PC2 and PC3 can help process pro-insulin[37]. Palizban et al have transfected rat small intestine K cells with the pGIP/Ins plasmid by the DOTAP liposome[38]. GIP can stimulate β cells to release insulin and promotes their regeneration[39,40]. Some reports have suggested that the antagonist of GIP can degrade the secretion of insulin remarkably[41]. As mentioned earlier, K cells could be one of the best target cells for the gene therapeutic treatment of type 1 diabetes due to its response to glucose and its resistance to cytokines and free radicals.
In conclusion, the human insulin gene can be transfected successfully by chitosan nanoparticles in vitro and in vivo, and is expressed efficiently in NIH3T3 cells and the gastrointestinal tracts of diabetes rats, indicating that chitosan is a promising non-viral vector for gene expression. To our knowledge, this study is to apply chitosan to the gene therapy of diabetes in living organisms as a vector. The benefits of using chitosan are its safety and painlessness. If it can be applied in clinics, it will be willingly accepted by patients. Although much work remains to be done, the rapid progress in insulin gene therapy provides an optimistic outlook for clinical applications to type 1 diabetes mellitus.
Acknowledgements
We are grateful to Dr Michael GERMAN from the Department of Hormone Research Institute at the University of San Francisco (San Francisco, CA, USA) for donating the pBAT16, hInsG1.M2 plasmid.
References
- Bell DS. Insulin therapy in diabetes mellitus: how can the currently available injectable insulins be most prudently and efficaciously utilised? Drugs 2007;67:1813-27.
- Dieterle C, Brendel MD, Seissler J, Eckhard M, Bretzel RG, Landgraf R. Therapy of diabetes mellitus. Pancreas transplantation, islet transplantation, stem cell and gene therapy. Internist (Berl) 2006;47:489-96.
- Samson SL, Chan L. Gene therapy for diabetes: reinventing the islet. Trends Endocrinol Metab 2006;17:92-100.
- Giannoukakis N, Trucco M. Gene therapy for type 1 diabetes. Am J Ther 2005;12:512-28.
- Zaia JA. The status of gene vectors for the treatment of diabetes. Cell Biochem Biophys 2007;48:183-90.
- Prud'homme GJ, Glinka Y, Khan AS, Draghia-Akli R. Electroporation-enhanced nonviral gene transfer for the prevention or treatment of immunological, endocrine and neoplastic diseases. Curr Gene Ther 2006;6:243-73.
- Chaszczewska-Markowska M, Stebelska K, Sikorski A, Madej J, Opolski A, Ugorski M. Liposomal formulation of 5-fluorocytosine in suicide gene therapy with cytosine deaminase for colorectal cancer. Cancer Lett 2008;262:164-72.
- Bajaj A, Kondaiah P, Bhattacharya S. Gene transfection efficacies of novel cationic gemini lipids possessing aromatic backbone and oxyethylene spacers. Biomacromolecules 2008;9:991-9.
- Kim HS, Kim JS, Lee YK, Koo KH, Park YS. An efficient liposomal gene delivery vehicle using Sendai F/HN proteins and protamine. Cancer Gene Ther 2008;15:214-24.
- Turner P, Petch A, Al-Rubeai M. Encapsulation of viral vectors for gene therapy applications. Biotechnol Prog 2007;23:423-9.
- Miyazaki M, Obata Y, Abe K, Furusu A, Koji T, Tabata Y, et al. Gene transfer using nonviral delivery systems. Perit Dial Int 2006;26:633-40.
- Ragusa A, García I, Penadés S. Nanoparticles as nonviral gene delivery vectors. IEEE Trans Nanobiosci 2007;6:319-30.
- Schmitz T, Bravo-Osuna I, Vauthier C, Ponchel G, Loretz B, Bernkop-Schnürch A. Development and in vitro evaluation of a thiomer-based nanoparticulate gene delivery system. Biomaterials 2007;28:524-31.
- Ren B, O'Brien BA, Swan MA, Koina ME, Nassif N, Wei MQ, et al. Long-term correction of diabetes in rats after lentiviral hepatic insulin gene therapy. Diabetologia 2007;50:1910-20.
- Fodor A, Harel C, Fodor L, Armoni M, Salmon P, Trono D, et al. Adult rat liver cells transdifferentiated with lentiviral IPF1 vectors reverse diabetes in mice: an ex vivo gene therapy approach. Diabetologia 2007;50:121-30.
- Mao HQ, Roy K, Troung-Le VL, Janes KA, Lin KY, Wang Y, et al. Chitosan-DNA nanoparticles as gene carriers: synthesis, characterization and transfection efficiency. J Control Release 2001;70:399-421.
- Shapiro AM, Lakey JR, Ryan EA, Korbutt GS, Toth E, Warnock GL, et al. Islet transplantation in seven patients with type 1 diabetes mellitus using a glucocorticoid-free immunosuppressive regimen. N Engl J Med 2000;343:230-8.
- Lau J, Nyqvist D, Köhler M, Berggren PO, Jansson L, Carlsson PO. Implantation site-dependent dysfunction of transplanted pancreatic islets. Diabetes 2007;56:1544-50.
- Li M, Inaba M, Guo KQ, Hisha H, Abraham NG, Ikehara S. Treatment of streptozotocin-induced diabetes mellitus in mice by intra-bone marrow bone marrow transplantation plus portal vein injection of beta cells induced from bone marrow cells. Int J Hematol 2007;86:438-45.
- Sutherland DE, Gruessner RW, Dunn DL, Matas AJ, Humar A, Kandaswamy R, et al. Lessons learned from more than 1,000 pancreas transplants at a single institution. Ann Surg 2001;233:463-501.
- Socci C, Orsenigo E, Zuber V, Caldara R, Castoldi R, Parolini D, et al. Triple arterial reconstruction improves vascularization of whole pancreas for transplantation. Transplant Proc 2006;38:1158-9.
- Vaca P, Berná G, Araujo R, Carneiro EM, Bedoya FJ, Soria B, et al. Nicotinamide induces differentiation of embryonic stem cells into insulin-secreting cells. Exp Cell Res 2008;314:969-74.
- Brenner S, Ryser MF, Whiting-Theobald NL, Gentsch M, Linton GF, Malech HL. The late dividing population of gamma-retroviral vector transduced human mobilized peripheral blood progenitor cells contributes most to gene-marked cell engraftment in nonobese diabetic/severe combined immunodeficient mice. Stem Cells 2007;25:1807-13.
- Timper K, Seboek D, Eberhardt M, Linscheid P, Christ-Crain M, Keller U, et al. Human adipose tissue-derived mesenchymal stem cells differentiate into insulin, somatostatin, and glucagon expressing cells. Biochem Biophys Res Commun 2006;341:1135-40.
- Fukushima M, Hattori Y, Tsukada H, Koga K, Kajiwara E, Kawano K, et al. Adiponectin gene therapy of streptozotocin-induced diabetic mice using hydrodynamic injection. J Gene Med 2007;9:976-85.
- Yoo HS, Mazda O, Lee HY, Kim JC, Kwon SM, Lee JE, et al. In vivo gene therapy of type I diabetic mellitus using a cationic emulsion containing an Epstein Barr Virus (EBV) based plasmid vector. J Control Release 2006;112:139-44.
- Lu YC, Sternini C, Rozengurt E, Zhukova E. Release of transgenic human insulin from gastric g cells: a novel approach for the amelioration of diabetes. Endocrinology 2005;146:2610-9.
- Cho YM, Lim JM, Yoo DH, Kim JH, Chung SS, Park SG, et al. Betacellulin and nicotinamide sustain PDX1 expression and induce pancreatic beta-cell differentiation in human embryonic stem cells. Biochem Biophys Res Commun 2008;366:129-34.
- Jafary H, Larijani B, Farrokhi A, Pirouz M, Mollamohammadi S, Baharvand H. Differential effect of activin on mouse embryonic stem cell differentiation in insulin-secreting cells under nestin-positive selection and spontaneous differentiation protocols. Cell Biol Int 2008;32:278-86.
- Serafimidis I, Rakatzi I, Episkopou V, Gouti M, Gavalas A. Novel effectors of directed and Ngn3-mediated differentiation of mouse embryonic stem cells into endocrine pancreas progenitors. Stem Cells 2008;26:3-16.
- D’Anneo A, Rood P, Bottino R, Balamurugan AN, He J, Giannoukakis N. Gene therapy for type 1 diabetes: is it ready for the clinic? Immunol Res 2006;36:83-9.
- Douglas KL, Piccirillo CA, Tabrizian M. Effects of alginate inclusion on the vector properties of chitosan-based nanoparticles. J Control Release 2006;115:354-61.
- Köping-Höggård M, Issa MM, Köhler T, Tronde A, Vårum KM, Artursson P. A miniaturized nebulization catheter for improved gene delivery to the mouse lung. J Gene Med 2005;7:1215-22.
- Koya V, Lu S, Sun YP, Purich DL, Atkinson MA, Li SW, et al. Reversal of streptozotocin-induced diabetes in mice by cellular transduction with recombinant pancreatic transcription factor pancreatic duodenal homeobox-1: a novel protein transduction domain-based therapy. Diabetes 2008;57:757-69.
- Okamoto R, Watanabe M. Molecular and clinical basis for the regeneration of human gastrointestinal epithelia. J Gastroenterol 2004;39:1-6.
- Cani PD, Holst JJ, Drucker DJ, Delzenne NM, Thorens B, Burcelin R, et al. GLUT2 and the incretin receptors are involved in glucose-induced incretin secretion. Mol Cell Endocrinol 2007;276:18-23.
- Cheung AT, Dayanandan B, Lewis JT, Korbutt GS, Rajotte RV, Bryer-Ash M, et al. Glucose-dependent insulin release from genetically engineered K cells. Science 2000;290:1959-62.
- Palizban AA, Salehi R, Nori N, Galehdari H. In vivo transfection rat small intestine K-cell with pGIP/Ins plasmid by DOTAP liposome. J Drug Target 2007;15:351-7.
- Cassidy RS, Irwin N, Flatt PR. Effects of gastric inhibitory polypeptide (GIP) and related analogues on glucagon release at normo- and hyperglycaemia in Wistar rats and isolated islets. Biol Chem 2008;389:189-93.
- Kuo P, Chaikomin R, Pilichiewicz A, O'Donovan D, Wishart JM, Meyer JH, et al. Transient, early release of glucagon-like peptide-1 during low rates of intraduodenal glucose delivery. Regul Pept 2008;146:1-3.
- McClean PL, Gault VA, Irwin N, McCluskey JT, Flatt PR. Daily administration of the GIP-R antagonist (Pro3)GIP in streptozotocin-induced diabetes suggests that insulin-dependent mechanisms are critical to anti-obesity-diabetes actions of (Pro3)GIP. Diabetes Obes Metab 2008;10:336-42.