Protective effects of L-arginine on pulmonary oxidative stress and antioxidant defenses during exhaustive exercise in rats1
Introduction
In recent years, it has been shown that L-arginine (L-Arg) serves as a precursor to the synthesis of NO and may participate in amino acid metabolism[1]. Most pharmacological actions of L-Arg are attributed to nitric oxide (NO), and NO has various physiological properties including vasodilatation, inhibition of platelet aggregation and neutrophil adhesion, scavenging superoxide (O2¯·) formation and suppression xanthine oxidase (XO)[2,3]. More recently, L-Arg has shown protective role against reactive oxygen radical (ROS) attack as a result of its interaction with O2¯· in vitro[4]. There is evidence that L-Arg is closely linked to protective properties against oxidative stress[5,6]. Further-more, L-Arg administration to rats prevented pulmonary neutrophil accumulation and preserved pulmonary endothelial function after endotoxin[7]. The XO activity of pulmonary artery endothelial cells decreased using L-Arg, suggesting that NO generated in the endothelial cells negatively regulates this enzyme[8].
Strenuous physical exercise may be associated with a 10- to 20-fold increase in whole body oxygen consumption and oxidative stress shown in both human and experimental animal studies[9,10]. The mitochondrial electron transport chain, XO and neutrophils of inflammatory response have been identified as major sources of intracellular free radical generation during exercise[11]. XO, a metalloflavoprotein, is an important source of ROS. The enzyme catalyzed the reduction of O2, leading to the formation of O2¯· and H2O2, and it has been proposed as a major mechanism of oxidative injury[12]. Reddy et al reported that XO activity and lipid peroxides increased in the lung tissue after exhaustive swimming exercise[13]. In addition, the activity of glutamine synthetase and reactive carbonyl derivatives enhanced in the rat lung during exhaustive running[14]. Similarly, activated neutrophils are known as a major source of ROS[15]. Neutrophils are capable of further generation of ROS through NADPH oxidase and producing hypochlorous acid from H2O2 through MPO reaction[16]. The NADPH oxidase system has been shown to be activated in response to various stimuli that can be provoked by strenuous exercise[17]. However, the lung tissue presents a significant first target of free radical species and gas exchange function of the respiratory system during exercise. Thus, the lung may be more vulnerable to oxidative damage as a result of exercise. Nevertheless, the pulmonary system has a series of defense mechanisms including SOD, CAT, GPX, GR, and GSH to protect the cell against these toxic oxygen metabolites, such as O2¯·, H2O2 and hydroxyl radicals (OH.). Strenuous exercise generated ROS seems to increase the activity of antioxidant enzymes and decrease GSH levels in the muscle and heart[18], possibly as a compensatory mechanism to cope with oxidative stress induced by exhaustive running.
However, the roles of L-Arg administration in exhaustive exercise-induced oxidative damage and inflammatory response in lung tissues are still not clear. Therefore, we studied whether L-Arg administration can protect lung tissue and have beneficial effects on ameliorating exercise-induced oxidative stress and reserve antioxidant defense capability in the lung of rats.
Materials and methods
Animals and treatment Adult male Sprague-Dawley rats (n=32), weighing 280–300 g and aged 8 weeks old, were purchased from the National Laboratory Animal Breeding and Research Center (Taipei). All rats were given standard rat chows and tap water ad libitum and housed at 23±2 °C on a 12:12-h dark-light cycle in the first week. This experiment was approved by the Fu-Jen Catholic University Animal Care and Usage Committee and followed the guidelines established by the National Laboratory Animal Breeding and Research Center in Taiwan. In the second week, in order to adapt to the experimental standard diet, all rats were fed AIN-93 purified diet[19]. Rats were then randomly divided into four groups: sedentary group with control AIN-93 diet (SC, n=8); sedentary group with AIN-93 containing 2% L-Arg diet (SC+Arg, n=8); exhaustive exercise with control AIN-93 diet (E, n=8); and exhaustive exercise with AIN-93 containing 2% L-Arg diet (E+Arg, n=8). Rats were fed diets for 30 d beginning from the third week. Both diets were identical in calorie content (Table 1)[19,20].
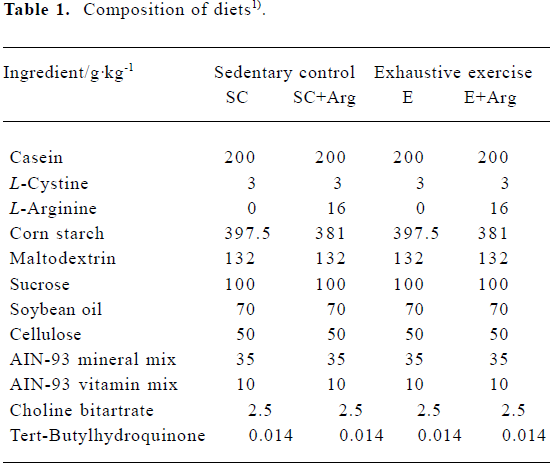
Full table
Exercise protocol All rats in the two exercise groups were introduced to treadmill running with 15–20 min exercise bouts at 15–30 m/min for 6 d to accustom them to running. In the formal run, the rats were warmed up for 15 min running at a speed of 15 m/min. They then progressed to running for 15 min at 20 m/min and 30 min at 25 m/min. They were then made to run to exhaustion at a final speed of 30 m/min, 10% grade, approximately 70%–75% VO2max[21]. The treadmill was equipped with an electric shock grid on the rear barrier to provide the animal with exercise motivation (using a T510E treadmill device; DR Instrument, Taipei). The point of exhaustion was determined by the loss of righting reflex when turned on its back. To eliminate diurnal effects, the experiments were performed at the same time (09:00–12:00 h). It is also well known that exercise performance is limited by the increase of body temperature during exercise. In order to eliminate this complicating factor, all running tests were conducted in an environmental chamber at 10–12 °C to reduce undue rise in body temperature caused by sustained exercise. All animals were anesthetized with ethyl ether and killed immediately after exhaustive exercise. Blood was collected through the abdominal aorta, and lung tissues were stored at -80 °C for further analysis. All chemicals used in this study were purchased from Sigma Chemical (St Louis, MO, USA) unless otherwise stated.
Plasma parameters Blood samples were centrifuged at 1400×g at 4 °C for 10 min. The supernatants (plasma) were used for determination of uric acid (UA) with an automatic analyzer (Hitachi 7170; Hitachi, Tokyo, Japan). In addition, nitrate/nitrite (NOx) levels in plasma were quantified by using the Griess reagent to measure nitrite ion concentration[22]. NOx concentrations in plasma were measured spectrophotometrically at 550 nm according to a previous study[23].
Total protein concentration of lung tissues In order to express the antioxidant enzyme activities per gram of protein, total protein concentration of tissue samples was spectrophotometrically estimated using a Bio-Rad DC protein assay kit (Cat N
Assay of xanthine oxidase activity in lung tissues XO (EC.1.2.3.2) activities of tissue samples were determined using the method outlined by Westerfeld et al[24]. A diluted sample was added to 0.1 mmol/L xanthine (dissolved in 50 mmol/L sodium phosphate buffer, pH 7.5). XO activity was measured at 25 °C on a Hitachi U-2000 Spectrophotometer at 290 nm for 3 minutes. A unit of XO activity was to form one mmmol of urate per minute at 25 °C. XO activity was expressed as U·g protein-1 for specific activity.
Assay of myeloperoxidase activity in lung tissues MPO (EC.1.11.1.7) activities of tissue samples were determined as a marker enzyme for measuring neutrophil accumulated in tissue samples, because correlates closely with the number of neutrophils present in the tissue[25]. MPO activity was determined using the method described by Schierwagen et al[26]. Fifty microlitre of diluted sample was added to 1 mL of mixed substrate containing 3 mmol/L hydrogen peroxide dissolved in 3,3',3,5'-tetramethylbenzidine (R&D Systems, Minneapolis, MN, USA). MPO activity was measured at 37 °C on a Hitachi U-2000 Spectrophotometer at 655 nm for 3 min. One unit of MPO activity was defined arbitrarily as the amount of enzyme necessary to catalyze an increase in absorbance of 1.0 at 655 nm per minute at 37 °C. MPO activity was expressed as U·mg protein-1 for specific activity.
Lipid peroxidation in plasma and lung tissues The malondialdehyde (MDA) concentrations of plasma and tissue samples were assessed colorimetrically at 586 nm using a commercial kit (Calbiochem 437634; Calbiochem-Novabio-chem, La Jolla, CA, USA). The concentration was expressed in µmol/L in plasma and in µmol·mg protein-1 in lung tissues.
Assay of superoxide dismutase activity in lung tissues SOD (E.C.1.15.1.1) activities of tissue samples were measured with a commercial kit (SD 125; Randox Laboratories, Antrim, UK). Fifty microlitre of diluted sample was added to 1.7 mL of mixed substrate (50 µmol/L xanthine and 25 µmol/L 2-(4-iodophenyl)-3-(4- nitrophenol)-5-phenyltetrazolium chloride, INT). Two hundred and fifty microlitre of XO were added to the mixture and SOD was measured at 37 °C on a Hitachi U-2000 Spectrophotometer at 505 nm for 3 min. Activity was expressed as U·mg protein-1 for specific activity.
Assay of catalase activity in lung tissues CAT (EC.1.11.1.6) activities of tissue samples were determined at 25 °C with Hitachi U-2000 Spectrophotometer UV-VIS spectrophotometer using the method described by Beers and Sizer[27]. A diluted sample was added to 59 mmol/L hydrogen peroxide (dissolved in 50 mmol/L potassium phosphate buffer, pH 7.0) and CAT was measured at 240 nm for 3 min. One unit of CAT activity was defined as the H2O2 degraded (mmol· min-1·mg protein-1). Activity was expressed as U·mg protein-1 for specific activity.
Assay of glutathione peroxidase activity in lung tissues GPX (EC.1.11.1.9) activities of tissue samples were determined with a commercial kit (RS 504; Randox Laboratories, Antrim, UK). Twenty microlitre of diluted sample was added to 1 mL of mixed substrate. Forty microlitre of cumene hydroperoxide (diluted in deionized water) was added to the mixture and GPX was measured at 37 °C with a Hitachi U-2000 Spectrophotometer at 340 nm for 3 min. Activity was expressed as U·mg protein-1 for specific activity.
Assay of glutathione reductase activity of lung tissues GR (E.C.1.6.4.2) activity of tissue samples was measured with a commercial kit (Calbiochem 359962; Calbiochem-Nova-biochem). Two hundred mmL of diluted sample was added to 400 µL of 2.4 mmol/L GSSG buffer (dissolved in 125 mmol/L potassium phosphate buffer, pH 7.5, 2.5 mmol/L EDTA). Four hundred mmL of 0.55 mmol/L NADPH (dissolved in deionized water) was added to the mixture and GR activity was measured at 340 nm for 5 min on a Hitachi U-2000 Spectro-photometer. The activity was expressed in mU·g protein-1 in tissue samples.
Assay of glutathione levels in plasma and lung tissues The concentrations of GSH in plasma and tissue samples were measured spectrophotometrically at 400 nm using a commercial kit (Calbiochem 354102; Calbiochem-Novabio-chem, La Jolla, CA, USA). The concentration was expressed in µmol/L in plasma and in µmol·mg protein-1 in tissue samples.
Statistical analysis Values were expressed as mean± SEM. To evaluate differences between the groups, two-way analysis of variance (ANOVA) with Fisher’s post hoc test was used. The SAS software (version 8.2, SAS Institute, NC, USA) was used to analyze all data. Differences were considered statistically significant when P<0.05.
Results
Bodyweight and endurance time On d 30, bodyweight in the groups SC, SC+Arg, E and E+Arg were 446±7, 446±10, 447±6, and 451±8 g, respectively. There was no significant difference in bodyweight between the 4 groups. The mean endurance time of treadmill running to exhaustion was 81±4 min for the group E and 87±5 min for the group E+Arg, which showed no significant difference between the 2 groups.
XO and MPO activities of lung tissues The XO and MPO activities of lungs in group E were significantly elevated by 113% and 55%, respectively, compared to those in the SC group (P<0.05). In contrast, the XO and MPO activities of lungs in the E+Arg group were significantly reduced by 65% and 24%, respectively, compared with those in the E group (P<0.05). Furthermore, there was no difference between the SC+Arg and E+Arg groups (Figure 1).
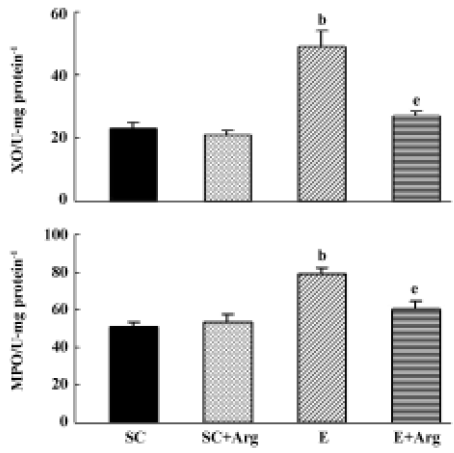
Uric acid and nitrite/nitrate concentrations of plasma The UA concentration of plasma in the E and E+Arg groups were significantly increased by 268% and 41%, respectively, compared with those in the SC and SC+Arg groups (P<0.05). Although, the UA concentration of plasma in the SC+Arg group was significantly increased compared with the SC group. This indicated that exogenous L-Arg may elevate UA in the plasma, and reduce UA excretion. However, L-Arg supplement might ameliorate UA concentration in plasma after exercise (Table 2). The NOx concentrations of plasma in the E and E+Arg groups were significantly increased by 93% and 82%, respectively, compared with those in the SC and SC+Arg groups (P<0.05). However, there was no difference between the SC and SC+Arg or E and E+Arg groups (Table 2).
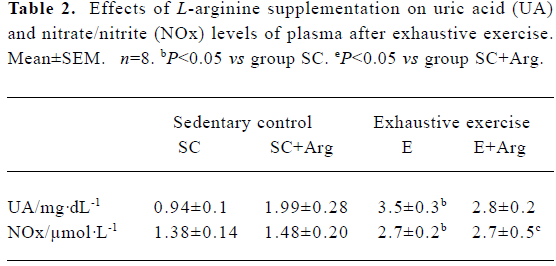
Full table
MDA levels of plasma and lung tissues The MDA levels of the plasma and lung showed no changes between SC+Arg and E+Arg groups. In contrast, the MDA levels of plasma and lung in the E group were significantly increased by 81% and 32%, respectively, compared to those in the SC group (P<0.05). There was a slight but not significant decrease in the MDA level of plasma in the E+Arg group compared to that in the E group (P>0.05) (Table 3).
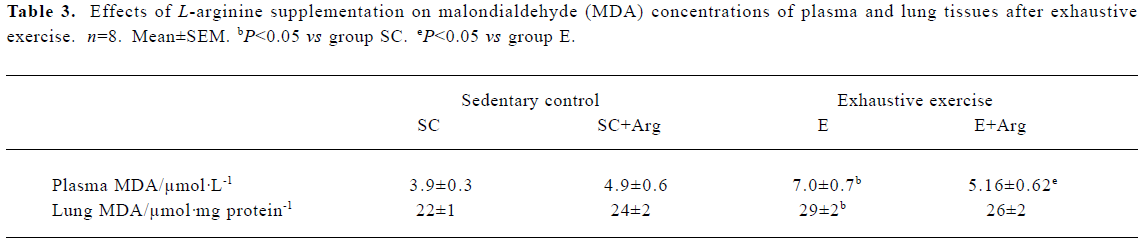
Full table
Antioxidant enzymes activities of lung tissues Table 4 shows the antioxidant enzyme activities. The E+Arg group had significantly higher GPX and CAT activities by 19% and 25%, respectively, than the group E (P<0.05), but showed no difference when compared with the SC+Arg group. Furthe-rmore, the GRD and SOD activities of the E group were significantly elevated by 44% and 96%, respectively, compared with those of the SC group (P<0.05), but they were significantly reduced by 35% and 58%, respectively, in the E+Arg group compared with the E group (P<0.05).
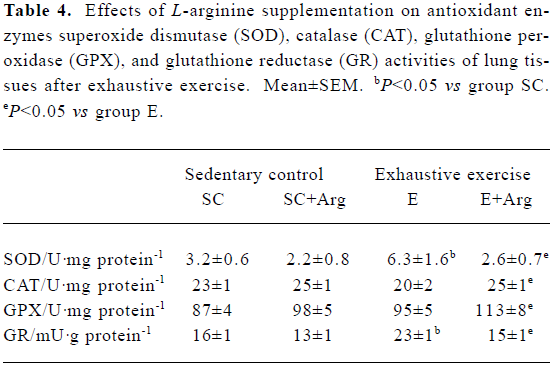
Full table
GSH concentrations of plasma and lung tissues The GSH concentration of plasma showed no change between SC+Arg and E+Arg groups. In contrast, the GSH concentration of plasma in the E group was significantly decreased by 25% compared with the SC group (P<0.05). Moreover, the GSH concentration of plasma in the E+Arg group was significantly increased by 31% compared with the E group (P<0.05). However, there was no difference in the GSH concentration of lungs in any group (Table 5).
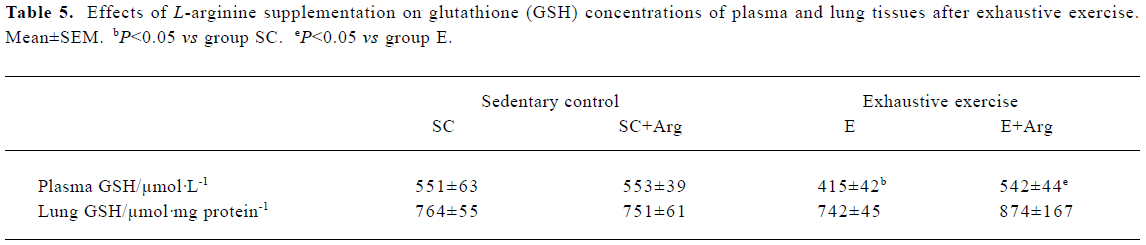
Full table
Discussion
The lung represents the front line of defense against a number of airborne toxicant and oxidant stress because it is directly exposed to higher oxygen tensions. In this study, we investigated whether P-Arg supplementation attenuates exhaustive exercise-induced pulmonary oxidative stress. More recently, there has been some evidence to show that strenuous exercise simulates the condition of heart I/R and may activate the XO pathway[28,29]. Hypoxanthine and UA are known to elevate in plasma during high intensity exercise[30]. Our results indicate that in exhaustive exercise with higher anaerobic components, the lactate concentrates in the plasma of the E group (E: 76±6 mg/dL vs SC: 55±4 mg/dL, P<0.05), but not in the E+Arg group. XO activity in the lung and plasma UA levels was also significantly increased in group E (P<0.05). Our data are consistent with previously published reports[30,31].
According to Yokoyama et al[32], the XO that is formed by the inadequate supply of oxygen to exercising muscles might have generated oxidative damage in other organs including the lung by causing an increase in the circulating level of XO, which binds in the lung to provide a pulmonary source of O2¯· . This is a possible mechanism that could explain increased XO activity in the lung under the current study. Lipid peroxidation causing tissue damage has been estimated by an extensively used indicator, such as MDA. The present study showed that after exhaustive running MDA levels (P<0.05) were significantly increased in the lung and plasma of the E group, compared to the SC group. However, the extent of the increase in pulmonary and plasma MDA levels in the E+Arg group was comparatively low as compared to the non-Arg group with exercise. This may suggest that the XO derived ROS exert some toxicant effect on lung tissue. In contrast, a smaller increase in XO and MDA concentration activity was observed in L-Arg fed rats in response to exhaustive exercise. This result in L-Arg fed rats could be responsible for protecting oxidative damage in the E+Arg group.
Numerous studies have shown that L-Arg is a precursor of NO, which can increase coronary vasodilation, scavenge various free radicals, inactivate Fe-S centers of XO and suppress XO activity as well as inhibit platelet and neutrophil adhesion to the endothelium[33,34]. Hence, the protective role of NO is probably a result of its ability to scavenge free radicals, reduce lipid peroxidation, and inhibit XO, which are in accordance with our results for the E+Arg group.
The current study showed that MPO activity in lungs from E group significantly increased after exhaustive exercise (P<0.05). However, there was no significant difference between E+Arg and SC groups. Hence, L-Arg supplementation to rats is successful in reducing post-exercise lung MPO activity and oxidative damage in lungs. These results are in accordance with previous studies about protecting the role of NO[12].
In the present study, our results indicate that both the E and E+Arg groups significantly increase plasma NOx after exhaustive exercise compared to the control. NO, however, is also a free radical and hence the toxicity of NO (excess product of NO) is markedly enhanced by its reaction with O2¯· from peroxynitrite (ONOO–), which is extremely cytotoxic[35]. However, Rubbo et al[36] recently reported that NO actually inhibits peroxynitrite-induced lipid peroxidation. This report demonstrated that NO will significantly enhance lipid peroxidation only when rates of NO production approach or are equivalent to rates of O2¯· production by XO. In the current study, we measure the effect of exhaustive exercise on the rate of NOx synthesis in plasma in both exercised groups. Although L-Arg supplementation effects animals subjected to exhaustive exercise on levels of NOx production, so the results cannot be distinctly different between both exercise groups in our study. This is likely to be related to the following two mechanisms. First, L-Arg serves as a precursor to the synthesis of NO that may act as an indirect antioxidant role by scavenging O2¯· and inhibiting XO activity from exercise-induced stress[37]. Our results suggest that L-Arg protection is generated through NO. NO has the capacity to inhibit reactive oxygen metabolites, including O2¯· and XO to prevent cellular damage, but in presence of the NO synthase inhibitor L-NAME, this protection was abolished, which suggests that the protection L-Arg is through the generation of NO[38]. Second, L-Arg has some protective roles against ROS attack possibly because of its direct chemical property interaction with O2¯· , thus suggesting a protective effect for L-Arg involving antioxidant mechanisms[4]. Obviously, the E group does cause a significant increase in XO and MPO activities, as well as MDA levels that are a result of oxidative stress in lung tissues. This means that the enhanced production of O2¯· by XO, may possibly be the combined work of higher level NO and O2¯· to form cytotoxic ONOO- after exhaustive exercise. In contrast with the E+Arg group, when NO concentrations are increased the protective role of NO is probably be a result of its ability to scavenge free radicals and possibly inhibit XO and inflame neutrophils. Therefore, this study suggests that the E+Arg group has higher levels of NO to inhibit ONOO– formation leading to lipid peroxidation and it is unlikely that ONOO– may have a role in this process.
In this study, SOD activity in the lung was significantly increased in response to exercise in the E group. As SOD is known to be an enzyme induced by its O2¯· substrate, the increase in the E group indicates an increased production of O2¯· . This finding was in agreement with Reddy et al[13], that SOD activity in the lung tissue significantly increased after strenuous swimming exercise. However, the SOD activity in the lung tissue of L-Arg-fed animals after exhaustive exercise was well below the control level, suggesting reduced generation of O2¯· and protection offered by L-Arg.
Furthermore, the CAT enzyme works on cooperating with GPX to scavenge excess H2O2 as well as lipid peroxides in response to oxidative stress. Under this situation, when O2¯· production through XO and neutrophils becomes an overwhelming compensatory mechanism, inadequate consequences take place. Possible mechanisms that could explain excess O2¯· are the inhibition of the activity of CAT by reduction of the Fe III center and inactivated GPX activity by oxidation of the selenium active site[39,40]. This interaction suggests SOD, CAT and GPX constitute a mutually supportive team of enzymes that provide a defense against the intermediaries of ROS. This study confirms Kono et al’s and Blum et al’s hypothesis, because overproducing O2¯· may speculate an inactive CAT and GPX activities and reciprocally influence these enzymes[39,40]. In contrast with the E+Arg group, the increased CAT and GPX activities seem to be an adaptive response of the lung to oxidative stress generated by exercise. An increase in activity of those enzymes is possibly a result of exposure to various oxygen toxicants. GR catalyzes the reduction of oxidized gluthione (GSSG) to GSH at the expense of NADPH and maintains high GSH/GSSG ratios in the cell. In the present study, GR activity of lung tissue was significantly increased after exhaustive running (P<0.05). The increase in GR activity of the lung suggested that the pulmonary antioxidant defense system of exercised rats reacted positively to combat ROS toxicity giving the tissue an adaptive response to oxidative stress. How-ever, our data showed that GR maintains the same levels in the L-Arg-fed animals at rest and after exercise, indicating that L-Arg supplementation has beneficial effects on attenuating the oxidative stress and maintaining GSH levels. GSH is the first line of defense against various types of chemicals[41]. As described, the GSH levels decreased in the liver and muscles after exhaustive exercise in rats[11]. In the current study, our data showed a depletion in pulmonary GSH and a significant decrease in plasma GSH in the E group compared with the SC group. However, in the E+Arg group the levels of GSH were restored, suggesting that the mechanism of protective action by L-Arg may involve maintenance of the thiol group of GSH. Our data correspond with previous reports that exhaustive exercise causes GSH oxidation in blood in both animals and in human beings[42,43].
Other possible mechanisms for an increase in GSH in lung tissue are that GSH synthesized primarily in the liver and was then transported to different organs of the body through the blood during prolonged exercise in order to maintain GSH homeostasis to cope with oxidative stress[44].
In conclusion, an acute bout of exhaustive exercise causes oxidative stress, tissue damage and antioxidant responses in the lungs. This study suggests that L-Arg supplementation can prevent elevations of XO and MPO activities in the lungs and can favorably influence pulmonary antioxidant defense systems after exhaustive exercise.
References
- Wu G, Morris SM Jr. Arginine metabolism: nitric oxide and beyond. Biochem J 1998;336:1-17.
- Kelly RA, Balligand JL, Smith TW. Nitric oxide and cardiac function. Circ Res 1996;79:363-80.
- Fukahori M, Ichimori K, Ishida H, Nakagawa H, Okino H. Nitric oxide reversibly suppresses xanthine oxidase activity. Free Radical Res 1994;21:203-12.
- Lass A, Suessenbacher A, Wolkart G, Mayer B, Brunner F. Functional and analytical evidence for scavenging of oxygen radicals by L-arginine. Mol Pharmacol 2002;61:1081-8.
- Lubec B, Hayn M, Kitzmuller E, Vierhapper H, Lubec G. L-Arginine reduces lipid peroxidation in patients with diabetes mellitus. Free Radic Biol Med 1997;22:355-7.
- Adawi D, Kasravi FB, Molin G, Jeppsson B. Oral arginine supplementation in acute liver injury. Nutrition 1996;12:529-33.
- Sheridan BC, Mcityre RC, Meldrum DR, Fullerton DA. L-Arginine prevents lung neutrophil accumulation and preserves pulmonary endothelial function after endotoxin. Am J Physiol Lung Cell Mol Physiol 1998;274:337-42.
- Cote CG, Yu FS, Zulueta JJ, Vosatka RJ, Hassoun PM. Regulation of intracellular xanthine oxidase by endothelial-derived nitric oxide. Am J Physiol Lung Cell Mol Physiol 1996;271:869-74.
- Sen CK. Oxidants and antioxidants in exercise. J Appl Physiol 1995;79:675-86.
- Ji LL. Oxidative stress during exercise: implication of antioxidant nutrients. Free Radic Biol Med 1995;18:1078-86.
- Ji LL. Antioxidants and oxidative stress in exercise. Proc Soc Exp Biol Med 1999;222:283-92.
- McCord JM. Oxygen-derived free radicals in postischemic tissue injury. N Eng J Med 1985;312:159-63.
- Reddy KV, Kumar TC, Prasad M, Reddanna P. Pulmonary lipid peroxidation and antioxidant defenses during exhaustive physical exercise: the role of vitamin E and selenium. Nutrition 1998;14:448-51.
- Radak Z, Nakamura A, Nakamoto H, Asano K, Ohno H, Goto S. A period of anaerobic exercise increases the accumulation of reactive carbonyl derivatives in the lungs of rats. Pflugers Arch 1998;435:439-41.
- Zhang SH, Wang SY, Yao SL. Antioxidative effect of propofol during cardiopulmonary bypass in adults. Acta Pharmacol Sin 2004;25:334-40.
- Tiidus PM. Radical species in inflammation and overtraining. Can J Physiol Pharmacol 1998;76:533-8.
- Suzuki K, Sato H, Kikuchi T, Abe T, Nakaji S, Sugawara K, et al. Capacity of circulating neutrophils to produce reactive oxygen species after exhaustive exercise. J Appl Physiol 1996;81:1213-22.
- Ji LL. Exercise and oxidative stress: role of cellular antioxidant systems. Exerc Sport Sci Rev 1995;23:135-66.
- Reeves PG, Nielsen FH, Fahey GC Jr. AIN-93 purified diets for aboratory rodents: final report of the American Institute of Nutrition ad hoc writing committee on the reformulation of the AIN-76A rodent diet. J Nutr 1993;123:1939-51.
- Lewis B, Langkamp-Henken B. Arginine enhances in vivo immune responses in young, adult and aged mice. J Nutr 2000;130:1827-30.
- Brooks GA, White TP. Determination of metabolic and heart rate responses of rats to treadmill exercise. J Appl Physiol 1978;45:1009-15.
- Green LC, Wagner DA, Glogowski J, Skipper PL, Wishnok JS, Tannenbaum SR. Analysis of nitrate, nitrite and [I5N] nitrate in biological fluids. Anal Biochem 1982;126:131-8.
- Schmidt HH, Warner TD, Nakane M, Forstermann U, Murad F. Regulation and subcellular location of nitrogen oxide synthases in RAW264.7 macrophages. Mol Pharmacol 1992;41:615-24.
- Westerfeld WW, Richert DA, Higgins ES. Further studies with xanthine oxidase inhibitors. J Biol Chem 1959;234:1897-900.
- Mullane KM, Kraemer R, Smith B. Myeloperoxidase activity as a quantitative assessment of neutrophil infiltration into ischemic myocardium. J Pharmacol Methods 1985;14:157-67.
- Schierwagen C, Bylund-Fellenius AC, Lundberg C. Improved method for quantification of tissue PMN accumulation measured by myeloperoxidase activity. J Pharmacol Methods 1990;23:179-86.
- Beers RF Jr, Sizer IW. A spectrophotometric method for measuring the breakdown of hydrogen peroxide by catalase. J Biol Chem 1952;195:133-40.
- Westing YH, Ekblom B, Sjodin B. The metabolic relation between hypoxanthine and uric acid in man following maximal short-distance running. Acta Physiol Scand 1989;137:341-5.
- Vina J, Gimeno A, Sastre J, Desco C, Asensi M, Pallardo FV, et al. Mechanism of free radical production in exhaustive exercise in humans and rats; role of xanthine oxidase and protection by allopurinol. IUBMB Life 2000;49:539-44.
- Hellsten-Westing Y, Sollevi A, Sjodin B. Plasma accumulation of hypoxanthine, uric acid and creatine kinase following exhausting runs of differing durations in man. Eur J Appl Physiol Occup Physiol 1991;62:380-4.
- Yamanaka H, Kawagoe Y, Taniguchi A, Kaneko N, Kimata S, Hosoda S, et al. Accelerated purine nucleotide degradation by anaerobic but not by aerobic ergometer muscle exercise. Metabolism 1992;41:364-9.
- Yokoyama Y, Beckman JS, Beckman TK, Wheat JK, Cash TG, Freeman BA, et al. Circulating xanthine oxidase: potential mediator of ischemic injury. Am J Physiol 1990;258:G564-70.
- Hassoun PM, Yn FS, Zulueta JJ, White AC, Lanzilla JJ. Effect of nitric oxide and cell redox status on the regulation of endothelial cell xanthine dehydrogenase. Am J Physiol Lung Cell Mol Physiol 1995;268:809-17.
- Nonami Y. The role of nitric oxide in cardiac ischemia-reperfusion injury. Jpn Circ J 1997;61:119-32.
- Beckman JS, Koppenol WH. Nitric oxide, superoxide, and peroxynitrite: the good, the bad and ugly. Am J Physiol Cell Physiol 1996;271:C1424-37.
- Rubbo H, Radi R, Trujillo M, Telleri R, Kalyanaraman B, Barnes S, et al. Nitric oxide regulation of superoxide and peroxynitrite-dependent lipid peroxidation. Formation of novel nitrogen- containing oxidized lipid derivatives. J Biol Chem 1994;269:26066-75.
- Pabla R, Buda AJ, Flynn DM, Blesse SA, Shin AM, Curtis MJ, et al. Nitric oxide attenuates neutrophil-mediated myocardial contractile dysfunction after ischemia and reperfusion. Circ Res 1996;78:65-72.
- Souza-Costa DC, Zerbini T, Metzger IF, Rocha JB, Gerlach RF, Tanus-Santos JE. L-Arginine attenuates acute pulmonary embolism-induced oxidative stress and pulmonary hypertension. Nitric Oxide 2005;12:9-14.
- Kono Y, Fridovich I. Superoxide radical inhibits catalase. J Biol Chem 1982;257:5751-4.
- Blum J, Fridovich I. Inactivation of glutathione peroxidase by superoxide radical. Arch Biochem Biophys 1985;240:500-8.
- Meister A, Anderson ME. Glutathione. Annu Rev Biochem 1983;52:711-60.
- Gohil K, Viguie C, Stanley WC, Brooks GA, Packer L. Blood glutathione oxidation during human exercise. J Appl Physiol 1988;64:115-9.
- Sastre J, Asensi M, Gasco E, Pallardo FV, Ferrero JA, Furukawa T, et al. Exhaustive physical exercise causes oxidation of glutathione status in blood: prevention by antioxidant administra-tion. Am J Physiol Regulatory Integrative Comp Physiol 1992;263:992-5.
- Ji LL, Fu R, Mitchell EW. Glutathione and antioxidant enzymes in skeletal muscle: effects of fiber type and exercise intensity. J Appl Physiol 1992;73:1854-9.