Prevention of diabetic microangiopathy by prophylactic transplant of mobilized peripheral blood mononuclear cells1
Introduction
Diabetic patients commonly suffer cardiovascular complications, including peripheral vascular disease. This is due to the concomitant acceleration of atherosclerosis and microvascular insufficiency[1]. Because of the absence of effective treatment, diabetic foot syndrome usually follows an inexorable course, and amputation is undertaken as a unique solution to unbearable symptoms at the end stage, which is as high as 28 000 per year in the USA[2]. Recently, therapeutic neovascularization based on a supply of angiogenic factors or angioblasts have been applied to ischemic limbs[3–6]. However, angiogenic factors such as vascular endothelial growth factor VEGF may fail to act when administered at advanced stages of the disease as a consequence of endothelial cell unresponsiveness and reduced availability of the downstream mediator nitric oxide[7]. Thus, a supply-side approach is deemed unsuccessful because while it provides the fuel (such as VEGF), it provides no ‘steering power’ to guide the reparative process[8]. Recently, Emanueli et al[9] demonstrated the progression of microvascular rarefaction in hind-limb skeletal muscles of diabetic mice and applied the prophylactic delivery of the human tissue kallikrein gene to ameliorate the peripheral diabetic complications. Therefore, it might be more effective to prevent diabetic microangiopathy than to rescue established ischemia in the late stage.
Since Asahara et al[10] first demonstrated the existence of circulating endothelial progenitor cells (EPC) in adult peripheral blood, the concept of adult vasculogenesis has been developed. Subsequently, studies showed that ex vivo-expanded EPC have utility as a ‘supply-side’ strategy for therapeutic neovascularization[11]. However, EPC in type I or II diabetes are dysfunctional[12,13], and their dysfunction may contribute to the pathogenesis of vascular complications in diabetic complications. In addition, widespread application of these cells in clinics is compromised as purifica-tion, and the cultivation of angioblasts (CD34+ cells) is expensive and time consuming. M-PBMNC are a rich source of angioblasts and can be easily obtained in a non-invasive manner. Our recent results provided pilot evidence that autologous transplantation of granulocyte colony-stimulating factor G-CSF–mobilized PBMNC represents a simple, safe, effective and novel therapeutic approach for diabetic critical limb ischemia[14]. However, the application of therapeutic angiogenesis for the prevention of microangiopathy in diabetes has been disregarded on the basis of current opinion that the strategy would fail in the absence of an ischemic milieu[9]. Since there is still no available permanent cure for diabetic critical limb ischemia at present[15,16], it has become more important to focus on the prevention of diabetic complications before the onset of diabetic vasculopathy, including non-healing ulcers, gangrene and nontraumatic limb amputation. The present study therefore investigates the local delivery of M-PBMNC as preventative treatment for peripheral microangiopathy in streptozotocin-induced nude mice. Here we report, for the first time, that the prophylactic local delivery of mobilized blood cells prevents diabetes-induced rarefication of capillary and arteriole density in nude mice adductor muscles. In addition, we found that injections of M-PBMNC attenuated apoptosis. These results were related to the survival, migration and incorporation of M-PBMNC into the murine vasculature in vivo, and M-PBMNC, which are rich in CD34+ cells, could provide abundant EPC to the diabetic nude mice.
Materials and methods
Cell preparation PBMNC, M-PBMNC were manipulated according to standard protocols approved by the Institutional Review Board of Chinese Academy of Medical Science and Peking Union Medical College, and written informed consent was obtained from all participating healthy volunteers. M-PBMNC were obtained from healthy donors who received treatment with G-CSF 600 µg/d by subcutaneous injection for 5 d. The purities of PBMNC were >97%, as determined by a differential leukocyte scattergram analysis (XE-2100, Sysmex, Kobe Japan. The depletion of the CD34+ fraction from MPBMNC was performed using CD34 magnetic microbeads (yi Biotec, GmbH, Germany) twice. The purity of the CD34+ cells in M-PBMNC before and after the deletion of the CD34+ cells were analyzed by flow cytometric assay FACS. Briefly, 1×106 mononuclear cells were incubated for 30 min at 4 °C in darkness with FITC-labeled monoclonal antibodies to CD34 (BD PharMingen, San Diego, California, USA). Isotype-matched mouse FITC-labeled immunoglobulin (BD PharMingen, San Diego, California, USA) served as controls. The cells were then washed twice and fixed in 1% polyformaldehyde, and quantitatively analyzed for 10 000 events by using FACSCalibur and CellQuest software (Becton Dickinson, San Diego, California, USA). M-PBMNC labeled with PKH2-GL (Sigma, St Louis, MO, USA) were cocultured with human umbilical vein endothelial cells HUVEC labeled with 1,1'-dioctadecyl-3,3,3',3'-tetramethyl-indocarbocyanine CM-DiL (Molecular Probe, USA) at 1:1 cell number on matrigel and observed 24 h later.
Animals All procedures were performed on male athymic nude mice (7–8 weeks, 16–20 g (Institute of Experimental Animal, Beijing, China) according to Peking Union Medical College’s Animal Care and Use Committee guidelines. The mice received streptozotocin 40 mg/kg ip (Sigma, USA) in 0.05 mol/L citrate buffer, pH 4.5, daily for 5 d[9]. Only the mice showing consistently elevated fasting glucose levels (250 mg/dL over 2 weeks) and overt glycosuria (>+ by test paper) were included in the study. Two weeks after the first positive glycosuria test was used as the date of the onset of diabetes; then, the diabetic mice anesthetized with 100 mg/kg sodium pentobarbital ip were injected intramuscularly over 8 sites 106 M-PBMNC or phosphate buffer saline PBS into the adductors using 26 gauge needles. To test the therapeutic potential for established microangiopathy, M-PBMNC were applied 42 d after the onset of diabetes, and capillary density was measured 14 d later. The capillary density, arteriole profile and apoptosis status in the adductor muscles harvested at selected time points were measured. Age-matched nondiabetic mice were used for reference. Mice that had lost more than 20% of their body weight during the study were not analyzed.
EPC culture and characterization PBMNC or M-PBMNC (106/mm2) were plated on culture dishes (BD, San Diego, CA, USA) coated with human fibronectin (Sigma, USA) and maintained in EC basal medium-2 supplemented with EGM-2 MV single aliquots (Clonetics, Cambrex, East Rutherford, NJ, USA). After 7 d in culture, the adherent cells (2×105–3×105) were immunostained with antihuman antibodies CD31, vWF, VE-Cadherin, KDR, CD34, CD45, CD3, and CD19. Isotype matched mouse immunoglobulin served as controls. The cells were quantitatively analyzed using FACSCalibur and CellQuest software (BD, San Diego, CA, USA). For the purpose of this study, the attached cells that showed an uptake of 1,1'-dioctadecyl-3,3,3',3' tetramethyl-indocarbo-cyanine labeled acetylated low-density lipoprotein (DiL-AcLDL) and binding of FITC-ulex europaeus agglutinin (UEA) were considered EPC[17]. Briefly, the cells were incubated with 10 µg/mL DiL-AcLDL (Molecular Probes, USA) at 37 °C for 4 h and fixed with 2% paraformaldehyde for 10 min. After washing, the cells were then incubated with 10 µg/mL FITC-UEA-1 (Sigma, USA) at 4 °C for 30 min. After washing with PBS twice, the nuclei of adherent cells were dyed with 4',6-Diamidine-2-phenylindole (DAPI. These cells were enumerated by examining 5 random microscopic fields (×200). At intervals following the onset of diabetes, the murine mononuclear cells isolated from 650 uL peripheral blood were separated by Histopaque-1083 (Sigma, USA) and cultured in 24-well plates coated with rat plasma vitronectin (Sigma, USA) in endothelial basic medium EBM-II media supplemented with 5% FBS (Clonetics, San Diego, CA, USA). After 4–5 d in culture, the EPC cells showing an uptake of DiL-AcLDL and binding of bandeiraea simplicifolia lectin 1 (BS-1 lectin, Vector Lab, California, USA) were detected and enumerated in 5 randomly selected fields (×200) under fluorescence micro-scopy.
Detection of apoptosis DNA fragmentation was determined by TUNEL assay. Deparaffinized 4 µm thick sections were stained with FITC-conjugated antibody (TUNEL, Roche, Switzerland) and counterstained with DAPI (Sigma, USA), or with streptavidin-conjugated peroxidase (with DAB as a chromogen). Sections were examined in a blinded fashion and TUNEL positive cell density was calculated as the number of apoptotic cells per square millimeter of section.
Histological assessment of muscle tissue Transverse cross-sections (4–5 µm) of each adductor muscle were cut. For each mouse, 5 sections perpendicularly to the adductor fiber were collected. In each group at each time point, 5 mice were euthanized to get adductor muscles. Deparaffinized 4 μm thick sections of adductor muscles were stained with anti mouse CD31, smooth muscle actin antibody (BD, San Diego, CA, USA) followed by incubation with FITC-conjugated secondary antibody. Five fields were randomly selected on the transverse sections for capillary counts and the capillary/muscle fiber ratio was also determined. Frozen sections of 5 µm thickness were counterstained with BS-1 lectin (Vector Lab) or CD31 antibody followed by incubation with FITC or TRITC-conjugated secondary antibodies. The capillary EC were counted under light microscopy to determine the capillary density. To ensure that capillary densities were not overestimated as a consequence of myocyte atrophy or underestimated because of interstitial edema, the capillary/muscle fiber ratio was determined. For identification of arterioles, the sections were stained with a mouse monoclonal anti-α-smooth muscle actin (Sigma, USA). Arteriole density per square millimeter of section (n art/mm2) was then calculated. Nonspecific immunoglobulins were used as the control for the above immunohistochemistry.
Detection of locally delivered M-PBMNC Seven, fourteen, and twenty-eight d after DiL-labeled M-PBMNC transplantation, the mice were euthanized with an overdose of pentobarbital, and ischemic tissue was obtained. Multiple frozen sections of 5 μm thick were prepared and examined under fluorescence microscopy and frozen sections of 50–60 µm were sequentially scanned using a confocal microscope (Leica Microsystems, GmbH, Germany). CD31 or BS-1 lectin was used to detect murine EC. Both the rhodamine and fluorescein filters were used for each image collected during the scanning process. The z series was converted into 40 projected images calculated from the original images. Individual projected images at each point were captured. 3-D picture was established by using LCS Lite software (Leica Microsystems, GmbH, Germany). For in vivo proliferation of implanted cells, Ki67 antibody was used (Sigma, USA) as described before[10].
Non-invasive in vivo imaging Non-invasive in vivo imaging by fluorescence was applied to track-injected cells in hind-limb muscles. Spectrally resolved images were taken from 500 to 720 nm at 10 nm intervals using a prototype of the CRI Maestro in vivo imaging system (23,24, Nuance, USA) and the resulting spectral data were unmixed using software provided with the system (Nuance, USA) .
Statistical analysis All results are expressed as mean±SD. Statistical significance of differences between groups was analyzed by ANOVA. If ANOVA indicated significant differences, the statistical value was determined according to the Bonferroni method. Differences between groups were determined with Student’s t-test. A P value <0.05 was significant.
Results
Prevention of microangiopathy by M-PBMNC Leukocyte scattergrams of peripheral blood indicated greater number of leukocytes were in circulation after G-CSF mobilization than those of the steady-state condition. Neutrophils and eosinocytes were excluded from the M-PBMNC fraction, while platelets were still included and were studied in combination with M-PBMNC (Figure 1A). By examination of the adductor muscle tissue, there was no significant change in the myofiber density during this period (data not shown). Rat anti-mouse CD31 monoclonal antibody was used to detected endothelial cells, which were stained in green fluorescence (Figure 1B). The capillary/fiber ratio progressively decreased as diabetes progressed, significantly more than in nondiabetic mice by d 70 (diabetic vs nondiabetes: 1.47±0.27 vs 2.04±0.10, P<0.05; Figure 1C). Local delivery of M-PBMNC resulted in the prevention of this capillary density decline (capillary/fiber ratio at d 70: 1.87±0.26 vs 1.47±0.15 in the PBS group, P<0.05; Figure 1D). In addition, the therapeutic potential of M-PBMNC could also be evident for the established microangiopathy at a later time (data not shown). As for arterioles, smooth muscle actin-positive cells were stained in green (Figure 2A). With the time from the onset of diabetes, the arteriole density of different sized luminal diameters decreased (diabetes vs nondiabetes: 2.91 vs 4.92 arterioles/mm2; Figure 2B). The preventive action of M-PBMNC was also evident at the arteriole level (Figure 2C), as M-PBMNC delivery ameliorated the decreased density of vessels of all luminal diameters at d 42 post injection. By TUNEL assay, apoptotic cells were stained with FITC or DAB (Figure 3A, 3B). With the diabetes onset, apoptotic cells increased significantly (Figure 3C). However, apoptosis was drastically reduced in the M-PBMNC-treated group (Figure 3D; TUNEL positive cells: M-PBMNC treated vs PBS treated vs non-treated groups: 6.2 vs 9.33 vs 9.5/mm2, P<0.05).
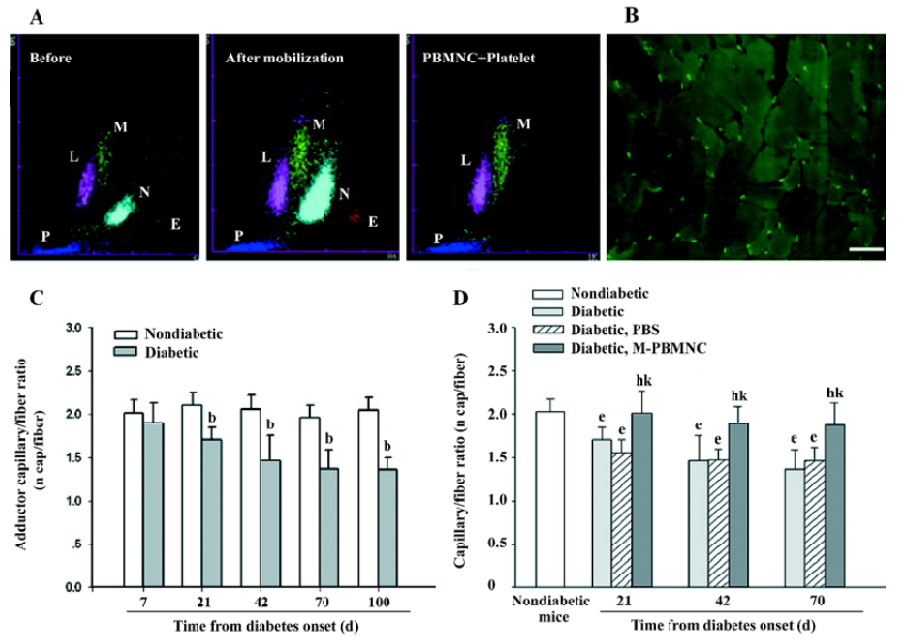
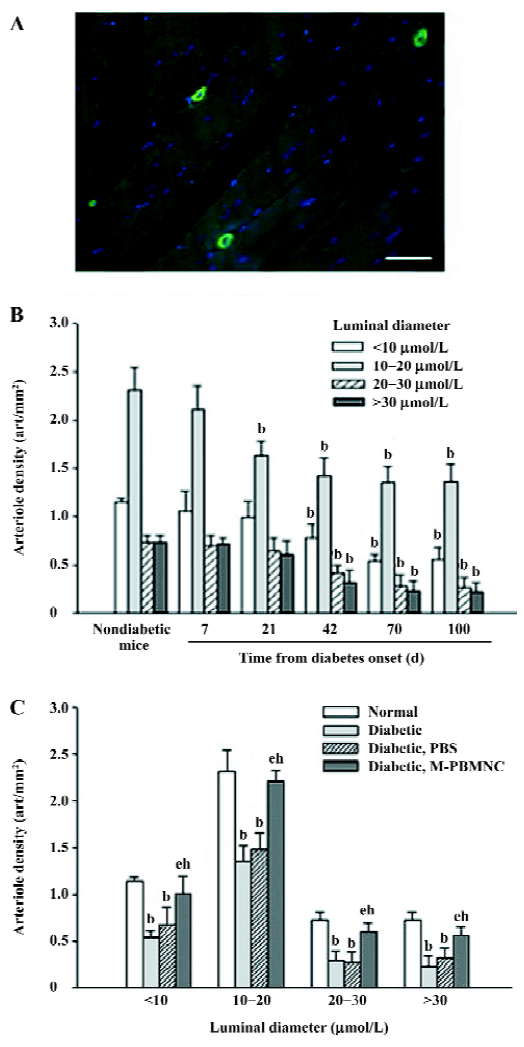
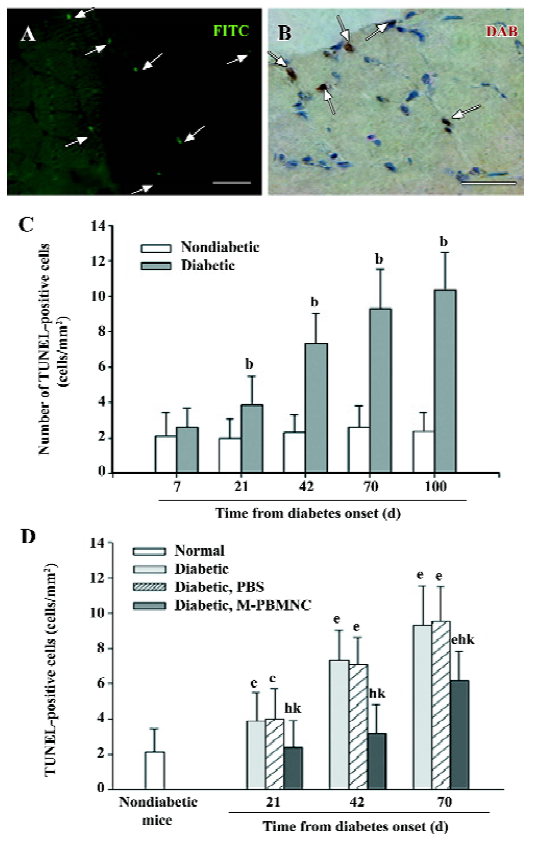
Participation of M-PBMNC in vasculature In vitro incorporation of PKH2GL-labeled M-PBMNC into DiL-labeled HUVEC networks on matrigel was identified (Figure 4A). In vivo fluorescence image showed some of the implanted cells survived at d 28 (Figure 4B), where a constellation of scattering transplanted cells was seen in the frozen sections of the tissue (Figure 4C). Transplanted cells sprouted from a place near the cell injection site indicated by *, and further migrated into the interstitial regions among preserved skeletal myocytes (indicated by M). Numerous labeled cells were incorporated and formed capillary-like networks, as some DiL-labeled cells (arrows) assembled near smooth muscle actin-positive arterioles (Figure 4D) while others colocalized with capillary cells immunostained for CD31 (green; Figure 4E). Figure 4F shows magnification of the colocalization of DiL-labeled M-PBMNC and murine capillaries (arrows). To further verify these findings, a serial scan of tissue using confocal microscopy spatially disclosed the incorporation of implanted cells into capillary network in 3 dimensions (Figure 4G, i–v in different scan plane of one tissue). These data suggested that the injected cells integrated into capillaries and surrounded the vessels as pericyte-like cells.
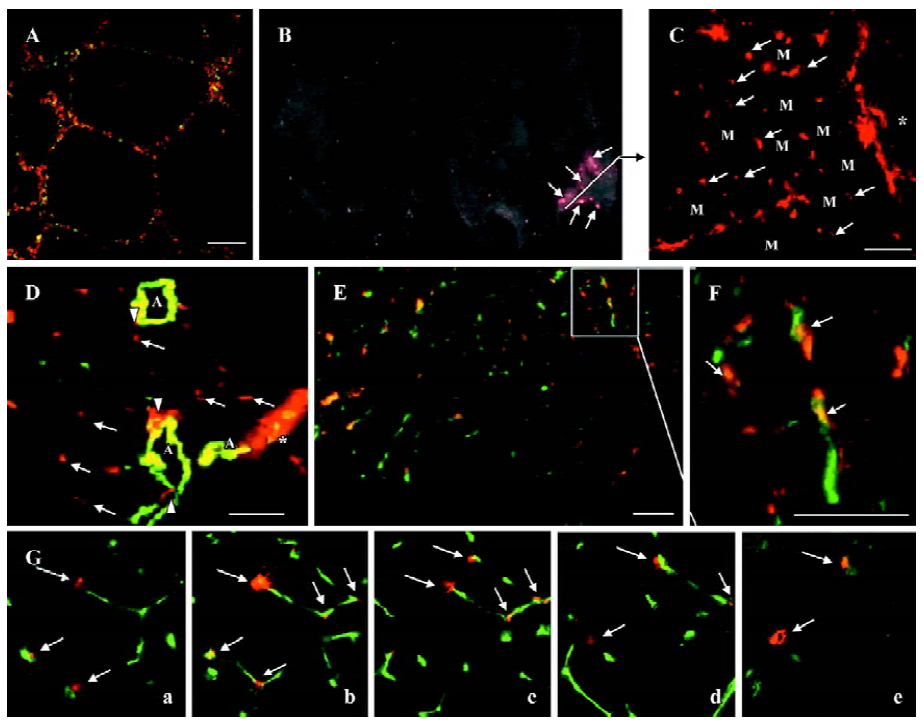
M-PBMNC incorporation in vivo The murine-attached cells were able to uptake DiL-AcLDL and stained with BS-1 lectin (Figure 5A). The murine EPC number decreased with the progression of diabetes (nondiabetic vs diabetic at d 70: 60.23±5.3 vs 40±4.2 in EPC number, P<0.05; Figure 5B), and this decline was represented by a decline in capillary density. Thus, the attenuation in microangiopathy is represented by a quantitative decline in EPC, and subsequently an impaired vasculogenesis. Both DiL-AcLDL and UEA-positive human attached cells and their nuclei were dyed with DAPI (Figure 5C). There were more EPC from M-PBMNC than PBMNC after 7 d in vitro culture (124±8.3 vs 75±10.2/×200 field, P<0.05), suggesting more angioblasts were available in M-PBMNC.
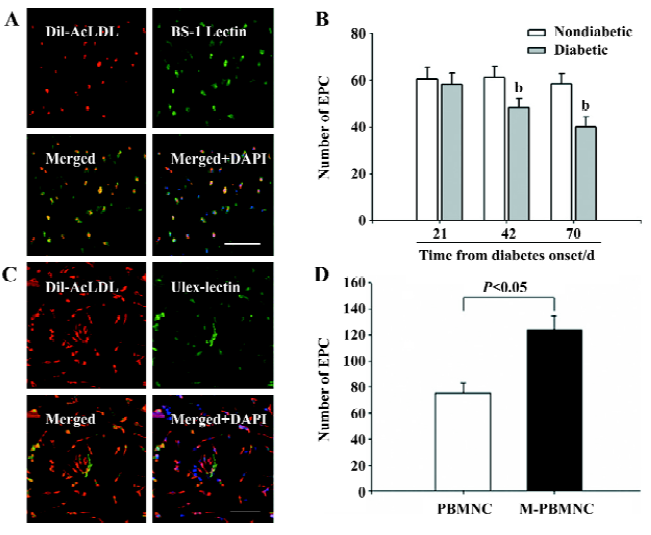
Discussion
In the present work, we found that the rarefication of capillary and arteriole can be prevented by prophylactic injections of M-PBMNC. Decreased apoptosis was found in M-PBMNC-treated tissue. Transplanted cells survived, migrated and incorporated into the murine vascular network. Thus, the early intervention to the still ‘healthy-appearing’ limbs of diabetic mice allowed prophylactic rescue from its later deteriorating progression. These findings are sufficiently important as we are at present carrying out clinical studies on preventative injections of autologous M-PBMNC to the ‘healthy-appearing’ legs of diabetic patients.
The balance between mechanisms that favor EC growth and vascular stabilization and that promote EC death and vascular regression is dysregulated in diabetes. Diabetes in the long term causes deteriorating microangiopathy, such as fewer vessels in limbs, ultimately leading to ischemia. In our study, the vascular rarefaction in limb skeletal muscles of diabetic mice is the result of an abnormally activated program that commits cells to premature death or apoptosis. Injections of M-PBMNC attenuated the apoptosis, as they migrated into interstitial regions of skeletal muscles, attached to and incorporated with the murine EC. Such physical cell-to-cell contact may be crucial for the survival of apoptotic EC, and can augment neovascularization by provoking murine EC proliferating[18]. Consequently, the decline of capillary densities was attenuated or even arrested.
In diabetic nude mice, the number of EPC progressively decreased, and this may suggest that decreased circulating EPC may contribute to the decrease in capillary rarefication and arteriole density. It is demonstrated that EPC were responsible for postnatal vasculogenesis in physiological and pathological neovascularization[19]. Under diabetic pathological conditions, therefore, we believed that vessel rarefication was due to impaired vasculogenesis caused by fewer circulating angioblasts that could be recruited for repairing and reconstituting injured vessels, including the replacement of those apoptotic EC. The transplantation of M-PBMNC directly brought a number of angioblasts into pathological foci where these progenitor cells can begin a reparative role. To supply EPC for impaired vasculature, we chose M-PBMNC instead of BM-MNC, since BM was not always available and is oftened influenced by chemotherapy, radiotherapy or heavy tumor infiltration. It is also difficult to collect a sufficient quantity of bone marrow for such transplantation in clinics. We first used transplantation of M-PBMNC instead of bone marrow cells to treat severe arteriosclerosis obliterans of lower extremities, and the therapeutic result was inspiring[20]. M-PBMNC have several advantages[21], and after mobilization, they may contain far more stem cells than steady-state BM[22,23]. Therefore, their transplantation has become an emerging and promising approach for diabetic complications[14].
It has been reported that EPC or angioblasts injected intramuscularly into ischemic hind-limbs of diabetic murine promoted blood perfusion recovery[24]. Locally delivered EPC or angioblasts were found to incorporate and formed capillary-like networks as well as tubular structures with a round lumen in vivo[18]. Our results showed that more EPC were available after mobilization and applicable to EPC-attenuated diabetic mice. These transplanted cells (CD34+ abundant cells) were found incorporated into the murine capillary networks and arterioles in vivo, which was in accordance with previous results[24]. Thus, the transplanted cells prevented the deterioration of microangiopathy by playing a supportive role to the murine EC.
Arterioles normally provide the largest part of hind-limb blood flow, and their remodeling in response to altered shear stress may ultimately lead to vascular collapse and regression. Since detrimental hemodynamic effects and tissue hypoxia derive especially from the drastic rarefaction of arterioles, we investigated the density of arterioles from the hind-limbs of mice at time intervals following the onset of diabetes. Arterioles of all sizes degenerate in diabetes, but progress in these defects could be prevented by the transplantation of M-PBMNC. This amelioration led to increased oxygen transport to myocytes, suggesting that diabetic microangiopathy is not an incurable condition.
Increased angiogenesis may result in abnormal blood vessel growth in ischemic heart, limbs and retina as paradoxical complications in diabetes patients[25]. Although systemic increases of mononuclear cells have been reported to benefit hind-limb ischemia, local M-PBMNC were intentionally chosen for the present study to avoid abnormal vasculo-genesis in other tissues, especially the retina. The absence of obvious protection in vascular deterioration in contralateral muscles further supported the effectiveness of local delivery of M-PBMNC. The lack of obvious transplanted cells in organs outside the injected hind-limb (Figure 4B) and histological assessment (data not shown) suggested the safety of our approach.
Here we used M-PBMNC from healthy volunteers for transplantation, but in clinical applications, we used autologous transplantation of M-PBMNC, which may have limitations[14]. Recently, we reported that M-PBMNC from diabetic patients were compromised in their efficacy in the treatment of diabetic ischemia[26]. It is likely that M-PBMNC from diabetic patients may also be impaired for prophylactic treatment in diabetic microangiopathy, since EPC from diabetic M-PBMNC were both fewer in quantity and dysfunctional in quality[12,13]. However, transplantation of diabetic M-PBMNC still promoted angiogenesis in ischemic limbs compared with that treated with PBS, albeit not as effective as that treated with nondiabetic M-PBMNC[26]. Clinically, allogenic transplantation of normal M-PBMNC may be more effective, but such transplanted cells may encounter rejection because of immunological problems. Therefore, autologous transplantation of M-PBMNC is still a good-albeit a compromised and not perfect-approach[27].
In addition to providing abundant angioblasts and to inhibiting apoptosis, M-PBMNC contain many angiogenic factors (such as VEGF) that have been proven to be effective in the ischemia model[28]. It was reported recently that the impaired ischemia-induced neovascularization in diabetes is associated with the dysregulation of a complex angiogenesis-regulatory network[29]. The disturbance of some angiogenic factors in the limb tissues was also found in diabetic microangiopathy[30]. In our experimental settings, we still can not rule out the possibilities that those mononuclear cells promoted EC proliferation and protected cell apoptosis via paracrine mechanism. The exact mechanism of how transplanted cells protect cells from apoptosis and how their secreted angiogenic factors influenced local milieu for EC survival and proliferation remains largely unknown and needs further investigation.
References
- Kannel WB, McGee DL. Diabetes and cardiovascular disease. The Framingham study. JAMA 1979;241:2035-8.
- Eckardt A, Kraus O, Kustner E, Neufang A, Schmiedt W, Meurer A, et al. Interdisciplinary treatment of diabetic foot syndrome. Orthopade 2003;32:190-8.
- Rajagopalan S, Shah M, Luciano A, Crystal R, Nabel EG. Adenovirus-mediated gene transfer of VEGF (121) improves lower-extremity endothelial function and flow reserve. Circulation 2001;104:753-5.
- Simons M, Annex BH, Laham RJ, Kleiman N, Henry T, Dauerman H, et al. Pharmacological treatment of coronary artery disease with recombinant fibroblast growth factor-2: double-blind, randomized, controlled clinical trial. Circulation 2002;105:788-93.
- Cao Y, Hong A, Schulten H, Post MJ. Update on therapeutic neovascularization. Cardiovasc Res 2005;65:639-48.
- Carmeliet P, Luttun A. The emerging role of the bone marrow-derived stem cells in (therapeutic) angiogenesis. Thromb Haemost 2001;86:289-97.
- Henry TD, Abraham JA. Review of preclinical and clinical results with vascular endothelial growth factors for therapeutic angiogenesis. Curr Interv Cardiol Rep 2000;2:228-41.
- Emanueli C, Graiani G, Salis MB, Gadau S, Desortes E, Madeddu P. Prophylactic gene therapy with human tissue kallikrein ameliorates limb ischemia recovery in type 1 diabetic mice. Diabetes 2004;53:1096-103.
- Emanueli C, Graiani G, Salis MB, Gadau S, Desortes E, Madeddu P. Prevention of diabetes-induced microangiopathy by human tissue kallikrein gene transfer. Circulation 2002;106:993-9.
- Asahara T, Murohara T, Sullivan A, Silver M, van der Zee R, Li T, et al. Isolation of putative progenitor endothelial cells for angiogenesis. Science 1997;275:964-7.
- Kalka C, Masuda H, Takahashi T, Kalka-Moll WM, Silver M, Kearney M, et al. Transplantation of ex vivo expanded endothelial progenitor cells for therapeutic neovascularization. Proc Natl Acad Sci USA 2000;97:3422-7.
- Loomans CJ, de Koning EJ, Staal FJ, Rookmaaker MB, Verseyden C, de Boer HC, et al. Endothelial progenitor cell dysfunction: a novel concept in the pathogenesis of vascular complications of type 1 diabetes. Diabetes 2004;53:195-9.
- Tepper OM, Galiano RD, Capla JM, Kalka C, Gagne PJ, Jacobowitz GR, et al. Human endothelial progenitor cells from type II diabetes exhibit impaired proliferation, adhesion, and incorporation into vascular structures. Circulation 2002;106:2781-6.
- Huang P, Li S, Han M, Xiao Z, Yang R, Han ZC. Autologous transplantation of granulocyte colony-stimulating factor-mobilized peripheral blood mononuclear cells improves critical limb ischemia in diabetes. Diabetes Care 2005;28:2155-60.
- Kugler CF, Rudofsky G. The challenges of treating peripheral arterial disease. Vasc Med 200:109-14.
- Williams DT, Harding KG, Price P. An evaluation of the efficacy of methods used in screening for lower-limb arterial disease in diabetes. Diabetes Care 2005;28:2206-10.
- Yang C, Zhang ZH, Li ZJ, Yang RC, Qian GQ, Han ZC. Enhancement of neovascularization with cord blood CD133+ cell-derived endothelial progenitor cell transplantation. Thromb Haemost 2004;91:1202-12.
- Murohara T, Ikeda H, Duan J, Shintani S, Sasaki K, Eguchi H, et al. Transplanted cord blood-derived endothelial precursor cells augment postnatal neovascularization. J Clin Invest 2000;105:1527-36.
- Asahara T, Masuda H, Takahashi T, Kalka C, Pastore C, Silver M, et al. Bone marrow origin of endothelial progenitor cells responsible for postnatal vasculogenesis in physiological and pathological neovascularization. Circ Res 1999;85:221-8.
- Huang PP, Li SZ, Han MZ, Xiao ZJ, Yang RC, Qiu LG, et al. Autologous transplantation of peripheral blood stem cells as an effective therapeutic approach for severe arteriosclerosis obliterans of lower extremities. Thromb Haemost 2004;91:606-9.
- Pecora AL, Lazarus HM, Jennis AA, Preti RA, Goldberg SL, Rowley SD, et al. Breast cancer cell contamination of blood stem cell products in patients with metastatic breast cancer: predictors and clinical relevance. Biol Blood Marrow Transplant 2002;8:536-43.
- Korbling M, Anderlini P. Peripheral blood stem cell versus bone marrow allotransplantation: does the source of hematopoietic stem cells matter? Blood 2001;98:2900-8.
- Jansen J, Hanks S, Thompson JM, Dugan MJ, Akard LP. Transplantation of hematopoietic stem cells from the peripheral blood. J Cell Mol Med 2005;9:37-50.
- Schatteman GC, Hanlon HD, Jiao C, Dodds SG, Christy BA. Blood-derived angioblasts accelerate blood-flow restoration in diabetic mice. J Clin Invest 2000;106:571-8.
- Lee IG, Chae SL, Kim JC. Involvement of circulating endothelial progenitor cells and vasculogenic factors in the pathogenesis of diabetic retinopathy. Eye 2006;20:546-52.
- Zhou B, Bi YY, Han ZB, Ren H, Fang ZH, Yu XF, et al. G-CSF-mobilized peripheral blood mononuclear cells from diabetic patients augment neovascularization in ischemic limbs but with impaired capability. J Thromb Haemost 2006;4:993-1002.
- Zhou B, Huang PP, Han ZC. Autologous transplantation of granulocyte colony-stimulating factor mobilized peripheral blood mononuclear cells improves critical limb ischemia in diabetes. Response to Fadini and Avogaro. Diabetes Care 2006;29:479-80.
- Li S, Zhou B, Han ZC. Therapeutic neovascularization by transplantation of mobilized peripheral blood mononuclear cells for limb ischemia: a comparison between CD34+ and CD34– mononuclear cells. Thromb Haemost 2006;95:301-11.
- Schiekofer S, Galasso G, Sato K, Kraus BJ, Walsh K. Impaired revascularization in a mouse model of type 2 diabetes is associated with dysregulation of a complex angiogenic-regulatory network. Arterioscler Thromb Vasc Biol. 2005;25:1603-9.
- Tanii M, Yonemitsu Y, Fujii T, Shikada Y, Kohno R, Onimaru M, et al. Diabetic microangiopathy in ischemic limb is a disease of disturbance of the platelet-derived growth factor-BB/protein kinase C axis but not of impaired expression of angiogenic factors. Circ Res 2006;98:55-62.